
- Chinese Optics Letters
- Vol. 23, Issue 2, 021601 (2025)
Abstract
1. Introduction
Modulating photoluminescence (PL) of light-responsive materials has aroused great interest because of its potential applications in super-resolution microscopy, displays, and optical memories[1–7]. These materials are typically constructed by the combination of a high PL material and a function-switchable material that can alternate between two states, with only one state capable of quenching the PL. One approach is the combination of quantum dots (QDs) with diarylethene (DAE) molecular photoswitches to modulate PL through alternating UV and visible light irradiation[8–16]. QDs as the PL materials feature excellent properties such as high PL quantum yields (PLQYs), flexible emission wavelength tunability, and narrow full width at half-maxima (FWHM) bandwidths[17–19]. DAE molecules can be interconverted between the ring-open isomer (o-DAE) and ring-closed isomer (c-DAE) upon alternating irradiation with UV and visible light[20–22]. DAE consists of two aryl groups connected by an ethene bridge, which can undergo a reversible photochemical reaction when exposed to UV and visible light. A cyclization process, where the ethene bridge forms a closed-ring structure, occurs upon UV light irradiation. The closed-ring form absorbs visible light, triggering a reverse reaction that breaks the ring and reverts it to the original open-ring structure. Their strong thermal stability and good reversibility make DAE molecules ideal switching materials for QDs[23]. Optically switching the PL of QDs can be achieved through Förster resonance energy transfer (FRET), triplet energy transfer[11] (TET), or charge transfer (CT), facilitated by spectral overlap or energy level alignment between QDs and c-DAEs, but not o-DAEs. Many optically switching PL systems have been reported by DAEs with CdSe/ZnS QDs[24], CdSe/CdS/ZnS QDs[10,25], CsPbX3 perovskite QDs[9], and PbS QDs[11]. However, the inherent toxicity of the above QDs limits their further application in biological and environmental detection. Therefore, it is essential to develop non-toxic PL switchable systems with a high PL on/off ratio (the PL intensity ratio between on and off states) and excellent reversibility under alternating irradiation cycles of different wavelengths.
Carbon quantum dots (CQDs) of less than 10 nm diameter, consisting of carbon atoms, have garnered significant attention in recent years as a non-toxic alternative to traditional semiconductor and perovskite QDs for bioimaging[26–33]. CQDs exhibit unique quantum confinement and edge effects, leading to the emission of visible light when excited. Moreover, functional groups on the surface of CQDs allow for tuning the PL range, binding to targets, and enhancing their stability and solubility in various solvents[34]. Although there are few reports on QD and DAE combinations for optically switching PL with a reasonable PL on/off ratio (up to 35)[12,35], synthesized CQDs exhibit a low PLQY (
Sign up for Chinese Optics Letters TOC. Get the latest issue of Chinese Optics Letters delivered right to you!Sign up now
Herein, we demonstrate a novel optically switchable PL material consisting of commercially available CQDs and DAEs via a simple mixing approach, as shown in Fig. 1(a). Efficient PL on/off switching is observed when the hybrid material is alternatively illuminated with 310 and 515 nm lights. The PLQY of CQDs is as high as 75% and unaffected in the presence of o-DAEs, whereas it can be significantly quenched by c-DAEs, achieving a PL on/off ratio up to 500. Our design also exhibits strong reversibility under 20 cycles of switching between UV and visible light irradiation. The mechanism of our efficient approach was investigated by PL lifetime measurement, temperature-dependent PL spectroscopy, and density functional theory (DFT) calculations. For the first time, to the best of our knowledge, we demonstrate that static quenching and inner filter (IF) effects between CQDs and c-DAE are the predominant processes enabling efficient PL switching.
Figure 1.PL modulation of CQD and DAE. (a) Schematic illustration and (b) photographs of PL modulation of the CQDs and DAEs in the on and off states. (c) Isomerization of o-DAE and c-DAE.
2. Experiment
2.1. Method
1,2-bis(2,4-dimethyl-5-phenyl-3-thienyl)-3,3,4,4,5,5-hexafluoro-1-cyclopentene (DAE) was purchased from TCI company. CQDs were purchased from Nanjing Muke Nanotechnology Co., Ltd. Both DAEs and CQDs had no ulterior depuration before use. Analytical reagent grade ethanol was purchased from AOPUSHENG chemical. All photophysical measurements were carried out in ethanol using 10 mm path quartz cuvettes.
2.2. Characterization
A transmission electron microscope (TEM, Tecnai G2, the Netherlands) was used to confirm the morphology of CQDs. Fourier-transform infrared (FTIR) spectra were acquired using KBr pellets on a Nicolet 6700 spectrometer. UV–visible absorption spectra were measured on a Cary 60 UV–vis NIR spectrophotometer. Steady-state and temperature-dependent PL spectra were recorded on a Cary Eclipse fluorescence spectrophotometer. PL lifetime measurements were carried out using time-correlated single photon counting (TCSPC), which was excited by a 400 nm laser diode (PicoQuant) and recorded by an MCP-PMT detector (10 000 counts, 2048 channels). For light irradiation, a UV analytic lamp (4.5 mW) was used for 310 nm light irradiation, and an LED light source (Zolix MLED 4-3) was used for 515 nm light irradiation (12 mW).
The PLQY of CQDs was determined by comparison of the absorption and PL spectra of fluorescein as a standard reference. Upon excitation at the same wavelength, the PLQY (
2.3. Calculation
DFT calculations of o-DAE and c-DAE were performed using the Gaussian 16 software package. Full optimization of the ground-state structure was performed by the hybrid functional B3LYP and basis set 3-21G(d).
3. Results
The properties of selected CQDs and DAEs were first investigated individually. A TEM image of CQDs reveals a uniform spherical morphology with an average diameter of 3–6 nm [Fig. 2(a)]. FTIR spectroscopy was used to detect the carboxyl functional group on the surface of the CQDs, and the spectrum is shown in Fig. 2(b). The peak at
Figure 2.Characterization of CQDs. (a) TEM image of CQDs. (b) FTIR spectrum of CQDs.
Figure 3.Absorption and PL spectra of CQDs and DAEs. (a) Absorption and PL spectra of CQDs (excited at 440 nm) in ethanol. (b) Absorption spectra of 50 µM DAE in ethanol. DAE was irradiated by 310 and 515 nm lights to the respective photostationary states.
The switching properties of DAEs were retained after mixing with CQDs. Figure 4(a) shows the absorption spectra of a solution of 2.5 µg/mL CQDs mixed with 50 µM DAEs in ethanol. Upon 310 nm UV irradiation, the appearance of absorption peaks in the visible region clearly indicates the formation of c-DAEs (red). Following irradiation at 515 nm, the absorption spectrum recovered to the initial state of o-DAEs (black). Adding o-DAEs into the solution of CQDs does not affect the PL characteristics, maintaining a PL peak at 570 nm and a PLQY of 75%, identical to CQDs alone. The PL of the mixed solution (2.5 µg/mL CQDs and 550 µM DAEs, approximately with the CQD/DAE mass ratio of 120) can be alternately switched between on and off states by exposure to UV and visible light, as shown in Fig. 4(b). Upon UV light irradiation, the PL intensity of CQDs is significantly reduced, achieving a 99.8% quenching efficiency and a PL on/off ratio of 500 (the normalized PL intensity at 570 nm compared to that of the closed-form 0.002). Irradiation at 515 nm fully restores the PL spectrum of CQDs. The PL on/off ratio in Fig. 4(b) is estimated to be 500, which outperforms all previously reported combinations of QDs and DAEs[9–11,25,35]. To assess the reversibility of our design, repeated cycles of light irradiation alternating between 310 and 515 nm were performed. The PL intensity at 570 nm was monitored after each light irradiation step, as shown in Fig. 4(c). No noticeable fatigue after 20 switching cycles demonstrates the excellent reversibility of our design. The modulation efficiency of the system (the PL intensity ratio of the difference between on and off states to the on state) reached 99.8%.
Figure 4.Light modulation of the absorption and PL spectra of CQDs mixed with DAEs. (a) Absorption spectra and (b) PL spectra of CQDs mixed with DAEs. (c) PL modulation switching over 20 cycles. DAE was irradiated by 310 and 515 nm lights to the respective photostationary states.
To investigate the mechanism of our well-performed optically switchable PL system, PL intensity and lifetimes of CQDs with various concentrations of DAEs were carried out. Since PL quenching occurs specifically between CQDs and c-DAEs, our discussion below only focuses on the quenching behavior of c-DAEs. The concentration of CQDs in the mixed solution was kept constant (2.5 µg/mL), while the concentration of c-DAEs varied from 0 to 100 µM, and the corresponding PL spectra and lifetimes are shown in Figs. 5(a) and 5(b). The PL intensity of CQDs decreases with increasing c-DAEs, whereas the PL lifetimes of CQDs only exhibit a slight decrease from 2.9 to 2.8 ns. Although the spectra overlap between PL of CQDs and the absorption of c-DAEs allow for dynamic quenching such as energy transfer to occur for the PL quenching. Dynamic quenching is a time-dependent collisional process, where the excited state of the PL donor encounters the PL acceptors and relaxes to the ground state through a non-radiative pathway[37]. Based on the PL lifetime measurements, the dynamic quenching contribution
Figure 5.PL and quenching behavior of CQDs and DAEs. (a) PL spectra of CQDs and (b) PL lifetime of the CQDs with c-DAEs (concentrations of 0, 10, 25, 50, and 100 µM in ethanol).
The spectral overlap between QDs and c-DAEs can also result in the IF effect. The IF effect can manifest in two ways: 1) primary IF where the excitation light is absorbed by components within the sample, reducing the detected PL intensity; 2) secondary IF where the emitted light is reabsorbed by components within the sample, further decreasing the PL intensity. None of the two IF processes can change the PL lifetime of the donor. Since c-DAE has nearly no absorbance at the excitation wavelength of 440 nm, the first process of the IF effect can be ignored. The IF effect causing the emission photon reduction in PL intensity
We hypothesized that the high quenching efficiency observed in CQDs and c-DAEs is also due to the static quenching. Static quenching occurs when the PL donors and acceptors bind in their ground states and form non-emissive complexes, and the static quenching cannot change the PL lifetime of the donor. Static and dynamic quenching can be distinguished by their behaviors at different temperatures. Higher temperatures increase the kinetic energy of the molecules, reducing the likelihood of the stable complex formation while increasing the likelihood of molecular collisions. Therefore, static quenching is less favorable at higher temperatures compared to dynamic quenching, such as FRET. PL spectra of QDs and c-DAEs were further investigated at low and high temperatures (0°C and 70°C). Figure 6(a) shows the Stern–Volmer plots at 0°C and 70°C with varied c-DAE concentrations. The quenching rate constant
Figure 6.Stern–Volmer plots of the mixture of CQDs and DAEs and geometrical structures of o-DAE and c-DAE. (a) Stern–Volmer plots for PL intensity of CQDs in 10 mm cuvette at 0°C and 70°C. (b) Side-views of the geometrical structures of o-DAE and c-DAE.
4. Conclusion
In conclusion, we have demonstrated an efficient optically switchable PL material consisting of commercially available CQDs and DAEs through a simple mixing approach. The PL of CQDs can be alternately switched on and off upon UV and visible light irradiation. The high PLQY of CQDs remains unaffected in the on state when DAEs are in the open form, while significant PL quenching occurs in the off state when DAEs convert to the closed form under UV light irradiation. Our design achieves a PL on/off ratio of up to 500 with excellent reversibility. Importantly, we investigated and revealed the mechanism behind this high performance. The PL quenching in the off state is primarily due to a strong IF effect and static quenching, with minimal contribution from dynamic quenching. These findings hold the potential of developing light responsive materials toward smart optoelectronics devices and imaging.
References
[36] I. N. Levine. Physical Chemistry(1983).
[37] M. Montalti, S. L. Murov. Handbook of Photochemistry(2006).
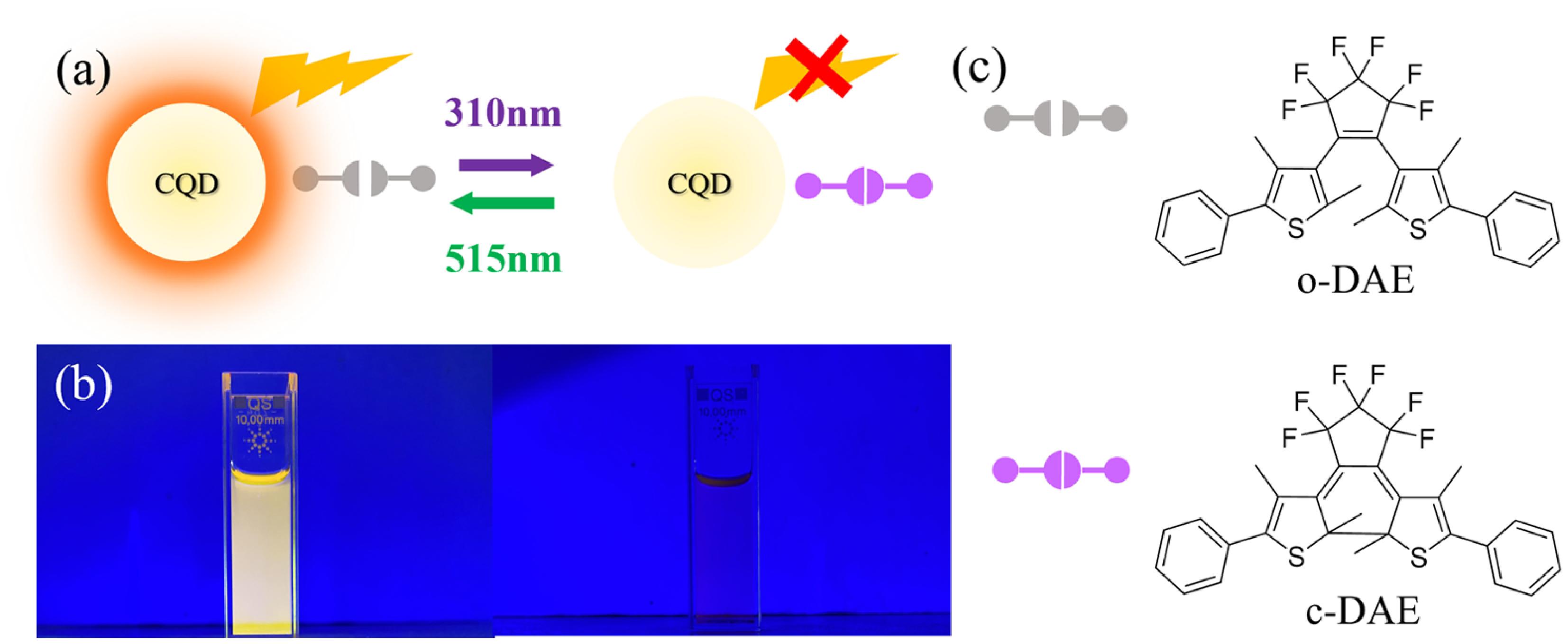
Set citation alerts for the article
Please enter your email address