
- Chinese Optics Letters
- Vol. 22, Issue 11, 110603 (2024)
Abstract
1. Introduction
As the next-generation intelligent integrated digital infrastructure, 6G will intersect and integrate with information technologies such as artificial intelligence, big data, and advanced computing, achieving deep coupling of communication with perception, computation, and control. It possesses typical characteristics such as ubiquitous connectivity, inclusive intelligence, multi-dimensional perception, comprehensive coverage, green and low-carbon, and inherent security[1,2]. Huawei company stated that 6G, like a massive distributed neural network, integrates communication, perception, and computing capabilities, deeply fusing the physical, biological, and digital worlds, truly ushering in a new era of “Everything Intelligent Connectivity”[3,4].
Research indicates that potential application scenarios of 6G include immersive extended reality, holographic communication, sensory interconnection, smart interaction, communication perception, and digital twin[5,6]. It can empower vertical industries such as the industrial Internet[7–9], Internet of Vehicles[10,11], smart cities[12,13], smart grids[14,15], smart agriculture[16], and smart healthcare[17,18], promoting the transition of humanity from the information age to the intelligent age, from the flat era to the three-dimensional era. The IMT-2030 (6G) Promotion Group defines the vision of 6G networks with two inherent characteristics (inherent intelligence and inherent security) and three integrations (integration of air, space, and ground; integration of communication and perception; and integration of computation and networking). In the future, the 6G transport network will be designed as an integrated computational and networking carrier, aiming to fully leverage the entrance advantages of the 6G network for users. It will use networking as the foundation, leveraging supercomputing/smart computing and capability resource pools to create differentiated computing services and realizing open service capabilities and collaborative operations of the integrated computing and networking system. This will establish a new type of computing and networking collaborative architecture with inherent computing power and inherent security[1].
With the growth of ubiquitous intelligent services such as holographic interaction, the metaverse, and smart transportation, as well as the increasing demand for AI-driven computation, there has been a significant surge in bandwidth requirements at the infrastructure resource layer. Optical network bandwidth continues to expand, with the backbone transmission network entering the era of 400G and long-distance 800G technology on the horizon[19,20]. Moreover, in the 5.5G and 6G eras, the rates of fronthaul, midhaul, and backhaul networks in mobile communication systems are also continuously increasing. However, with the improvement of optical communication rates, increased optical power, and extended transmission distances, the accumulation of nonlinear effects has caused significant damage to optical communication signal transmission, becoming one of the major factors restricting system performance. Hence, research on advanced optical modulation formats has been a key focus of researchers[21–23]. The frequency shift keying (FSK) modulation format, due to its ability to carry different service signals through different frequencies with constant power and high nonlinear resistance, has gained widespread attention in recent years[24–27]. However, existing research on single-channel systems mainly focuses on rates below 40 Gbps[25–27]. This study proposes an advanced alternating polarization chirped return-to-zero frequency shift keying (Apol-CRZ-FSK) modulation format for 100 Gbps optical communication systems that can meet the integrated computing and networking carrying requirements of future 6G. Based on the FSK modulation format, the chirp amount is changed to offset the impact of dispersion and enhance the dispersion tolerance of the signal. In addition, the orthogonal polarization states of adjacent bit optical signals reduce their power interactions and improve the nonlinear tolerance.
2. System Architecture and Basic Principle
In the Outlook on 6G Network Architecture released by the 6G Promotion Group at the global 6G Development Conference in 2023 for the first time, the 6G network architecture is divided into three layers, mainly including the infrastructure resource layer, network function layer, and application and openness layer. As shown in Fig. 1, the application and openness layer provides multidimensional capability opening for holographic interaction, industrial Internet, smart transportation, smart healthcare, the metaverse, and other application scenarios of the 6G era through services such as data, computing, perception, AI, connectivity, and security. The network function layer provides unified scheduling of multiple elements for the upper-layer applications and openness through connection, computation, AI, data, perception, and other functions. However, all these functionalities rely on the supply of various types of basic resources in the infrastructure resource layer, which mainly includes infrastructure such as computing power and networking resources. In the era of 6G, networking and computing are deeply integrated, with networking promoting computing and computing supporting networking. Networking and computing perceive each other, coordinate with each other, realize real-time and accurate computing resource discovery, realize flexible and dynamic service scheduling, provide ubiquitous computing and services, achieve rational allocation of computing resources and user unawareness, empower consistent user experiences, improve the efficiency of network and computing resource utilization, and realize efficient collaboration among cloud, edge, network, and computing.
Figure 1.6G integrated computing and networking architecture. (a) Apol-CRZ-FSK signal transmitter. (b) Apol-CRZ-FSK signal receiver.
In the infrastructure resource layer of the 6G integrated computing and networking architecture, the Apol-CRZ-FSK modulation format can be applied in 100 Gbps optical cross connect (OXC) optical transmitters and receivers. As shown in Fig. 1(a), the optical signal emitted by the laser CW1 of the Apol-CRZ-FSK signal transmitter is sent to the Mach–Zehnder modulator 1 (MZM1), which operates in a push–pull state with the amplitude of the RF driving voltage set to half the half-wave voltage, and the direct current (DC) bias is set at the zero-crossing point of the transmission curve. At this time, the carrier suppression can be achieved by modulating the MZM1 to obtain the required two wavelengths, and the wavelength interval is twice the frequency of the RF signal. The resulting signal is then sent to a phase modulator for differential phase shift keying (DPSK) modulation of the two different frequency optical signals, generating two DPSK signals that are subsequently transmitted to MZM2.
Therefore, the optical field and optical intensity
Following this, the signal processing by a 1-bit delay Mach–Zehnder delay interferometer (MZDI) generates a return-to-zero frequency shift keying signal carrying optical pulses at each bit interval, which is then transmitted to the phase modulator. The phase modulator is utilized to control the chirp, producing a CRZ-FSK signal that is subsequently sent to the polarization alternator. The CRZ-FSK signal is split into two paths by the polarization beam splitter (PBS), with each path containing orthogonally polarized light with equal intensity. One path of polarized light directly enters the polarization beam combiner (PBC), while the other path of polarized light passes through the second phase modulator to impose modulation signals with phases of 0 and
3. Research on System Simulation
This research employs VPItransmissionMaker Optical Systems version 9.3 for the comprehensive simulation of the transmission system, with the signal transmission rate set at 100 Gbps. On the transmission line of the high-speed optical communication system, an erbium-doped fiber amplifier (EDFA) is initially employed to amplify the signal power, facilitating its long-distance transmission. The dispersion compensation fiber can be placed before the single-mode fiber (SMF), known as pre-compensation, after the SMF as post-compensation, or evenly placed before and after the SMF as hybrid compensation. On the receiving side, the signal should be amplified by an EDFA to compensate for the optical power loss during long-distance transmission and then received and demodulated by a direct detection receiver. Table 1 displays the parameter settings for the fiber link.
SMF | DCF | |
---|---|---|
Dispersion (ps nm−1 km−1) | 16 | −90 |
Attenuation (dB/km) | 0.2 | 0.5 |
Nonlinear index (m2/W) | 2.6 × 10−20 | 4 × 10−20 |
Core area (m2) | 8.0 × 10−11 | 5.5 × 10−11 |
Dispersion slope (ps nm−2 km−1) | 0.08 | −0.45 |
PMD coefficient (ps/km) | 10−13/31.62 | 10−13/31.62 |
Table 1. Parameters of Transmission Line
This study compared the transmission performance differences of 100 Gbps Apol-CRZ-FSK, CRZ-FSK, and differential quadrature phase shift keying (DQPSK) signals with pre-, post-, and hybrid dispersion compensation.
The relationship for the Q-factor variations of three different modulation signals under the same conditions as the launch power ranged from
Figure 2.Performance of 100 Gbps Apol-CRZ-FSK signals for different compensation schemes. (a) Pre-compensation, (b) hybrid-compensation, (c) post-compensation.
Figure 3.Transmission performance of 100 Gbps Apol-CRZ-FSK signals at the longest single span distance of 175 km.
Figure 4.Performance of 100 Gbps CRZ-FSK signals under different compensation schemes. (a) Pre-compensation, (b) hybrid-compensation, (c) post-compensation.
As the launch power gradually increases indicated in Figs. 2(a)–2(c), the Q-factor of the signal at the same transmission distance gradually increases, reaching its maximum value, and then it gradually decreases. When the launch power is
Transmission performance of 100 Gbps CRZ-FSK signals under three compensation schemes is shown in Figs. 4(a)–4(c). Similar to the transmission curve of Apol-CRZ-FSK signals, the Q-factor of the three compensation schemes is around 6 dB under the condition of 90 km and the launch power of
Figures 5(a)–5(c) illustrate the transmission performance of 100 Gbps DQPSK signals. The Q-factor of the three compensation schemes is around 4 dB while the signal transmission distance is 90 km and the launch power is
Figure 5.Performance of 100 Gbps DQPSK signals under different compensation schemes. (a) Pre-compensation, (b) hybrid-compensation, (c) post-compensation.
Figure 6 compares and analyzes the relationship between the bit error rate (BER) and the launch power for three signals at 80 km. When the launch power is 13 dBm, the 100 Gbps Apol-CRZ-FSK signal exhibits the best BER performance, significantly better than that of the other two signals.
Figure 6.BER performance of 100 Gbps Apol-CRZ-FSK, CRZ-FSK, and DQPSK signals at 80 km transmission.
This study investigated the relationship between the launch power and the Q-factor of 100 Gbps Apol-CRZ-FSK, CRZ-FSK, and DQPSK signals at these typical span transmission distances. As shown in Fig. 7, when the launch power is
Figure 7.Transmission performance of 100 Gbps Apol-CRZ-FSK, CRZ-FSK, and DQPSK signals at single-span distances of 80 km, 100 km, and 120 km.
As the launch power gradually increases, the Q-factors of the three signals also increase gradually. After reaching a peak value, nonlinear effects become the main factor affecting system performance with further increases in power and distance. The Q-factor gradually decreases as the launch power increases and the distance lengthens, with larger nonlinear effects leading to greater differences in Q-factor among the three signals. Figure 7 shows that the Q-factor of Apol-CRZ-FSK signals is 7.5 dB and 9.6 dB higher than that of CRZ-FSK and DQPSK signals, respectively, while the best transmission performance conditions are achievable at 80 km. Under the best transmission performance conditions achievable at 100 km, the Q-factor of Apol-CRZ-FSK signals is 12.9 dB and 16.5 dB higher than that of CRZ-FSK and DQPSK signals, respectively. The Q-factor of Apol-CRZ-FSK signals is 10.2 dB and 13.6 dB higher than that of CRZ-FSK and DQPSK signals, respectively, at 120 km.
The performance of 100 Gbps Apol-CRZ-FSK signals after transmission over multiple span distances is analyzed in Fig. 8. Each span consists of a segment of SMF, a dispersion compensating fiber, and an EDFA used to compensate for the signal’s long-distance transmission power loss. The number of spans ranges from 5 to 100, and each span distance varies gradually from 30 km to 120 km. The launch power ranges from
Figure 8.Performance of 100 Gbps Apol-CRZ-FSK signals over multi-span distances.
When the SMF length of each span is 60 km, the optimal transmission performance is achieved with an launch power of 7 dBm, resulting in a Q-factor of 9 dB for 600 km transmission; for signal transmission over 960 km, the optimal transmission performance is achieved with a launch power of 9 dBm.
4. Discussion and Conclusion
This study proposes an advanced modulation format Apol-CRZ-FSK, which meets the requirements of integrated computing and networking for future 6G. Based on the FSK modulation format, the chirp amount is changed to offset the impact of dispersion and enhance the dispersion tolerance of the signal. In addition, the orthogonal polarization states of adjacent bit optical signals reduce their power interactions and improve the nonlinear tolerance. An incoherent single-ended detection method can be directly employed on the receiver side for frequency discrimination, enabling signal demodulation reception using a direct detection receiver, which is simple, practical, and cost-effective. Furthermore, comprehensive comparison and analysis of the transmission performance of 100 Gbps Apol-CRZ-FSK, CRZ-FSK, and DQPSK signals are conducted under identical conditions. The research indicates the high nonlinearity resistance capability exhibited by Apol-CRZ-FSK, highlighting its superior transmission performance.
References
[1] . 6G Computing Network Integrated Bearing Requirements and Architecture Research Report(2023).
[2] . 6G Typical Scenarios and Key Capabilities White Paper(2022).
[4] W. Tong, P. Zhu. 6G: The Next Horizon: From Connected People and Things to Connected Intelligence(2021).
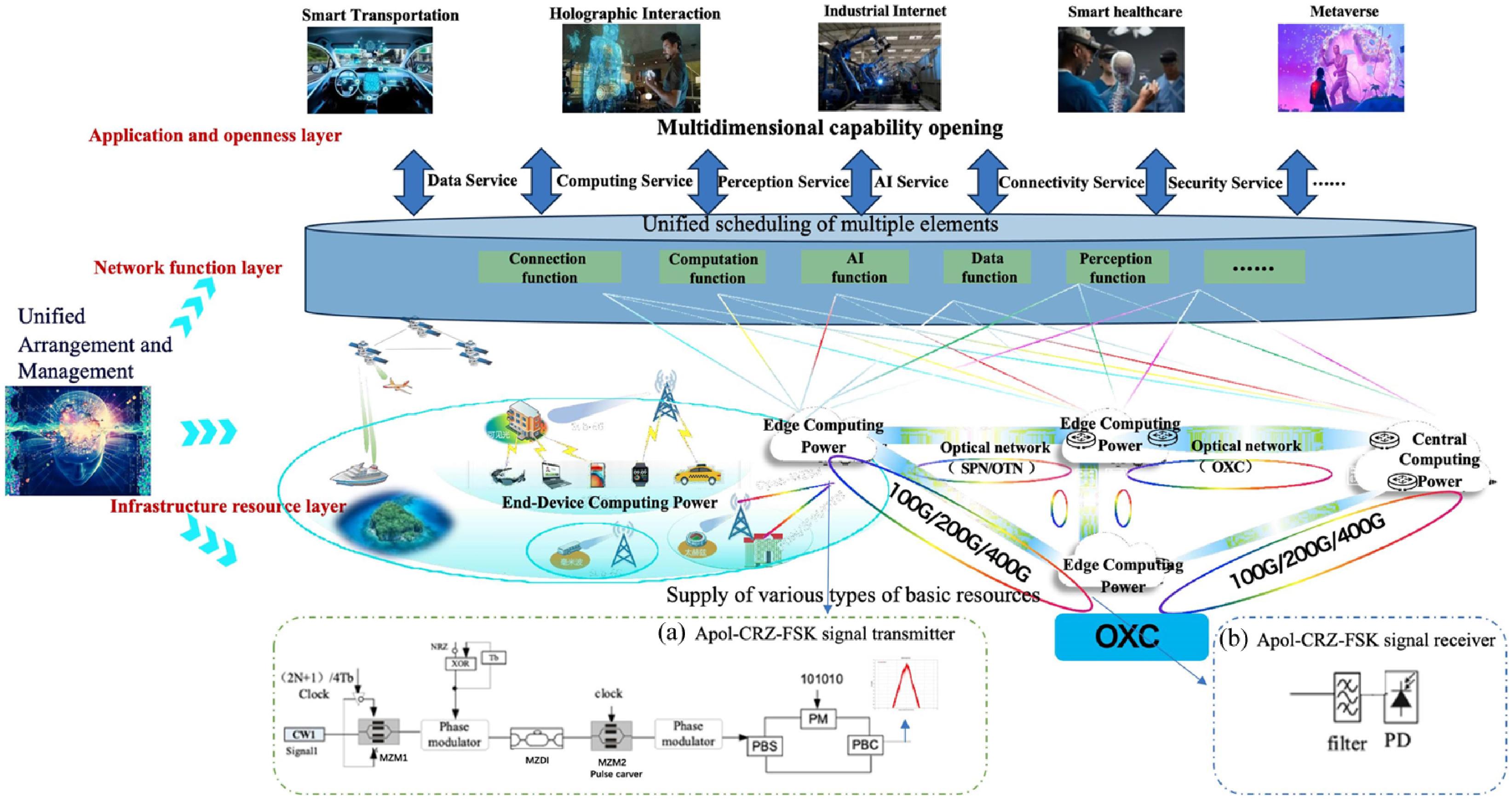
Set citation alerts for the article
Please enter your email address