
- Advanced Imaging
- Vol. 1, Issue 3, 032002 (2024)
Abstract
Keywords
1. Introduction
Photoacoustic (PA) imaging (PAI) is a biomedical imaging technique that exploits the PA effect to visualize the contrasts of optical absorption among biological tissues. The PA effect refers to the instantaneous thermal expansion of optically absorptive targets when they are illuminated by a laser pulse, followed by the generation of ultrasound (US) waves[1,2]. A PAI system detects these waves using US transducers and then uses the signals to reconstruct PA images that show the targets in the tissue[3]. PAI provides deeper imaging depth than optical imaging because US waves scatter less than light does within biological tissues. The major targets of biomedical PAI include hemoglobin (Hb) and melanin, using methods that exploit their high optical absorption in the visible and near-infrared (NIR) spectral ranges[4–7]. Therefore, PAI can provide detailed images of vascular structures or melanin distribution. To expand PAI applications, PA images can be obtained using other spectral ranges, such as ultraviolet (UV) or even x-rays[8,9]. Using multi-wavelength laser illumination, PAI can separate the mixed PA signals from various chromophores by applying spectral unmixing techniques to their unique absorption spectra[10–12]. Spectral unmixing of oxy-hemoglobin (
These advantages make PAI suitable for various fields of pre-clinical research such as neuroimaging, ophthalmic imaging, skin imaging, cancer imaging, and cardiovascular or peripheral vascular imaging. Neuroimaging applications of PAI provide a high-resolution mapping of
In some areas, PAI is also being applied to clinical studies as well as pre-clinical research[37–39]. In cancer imaging, PAI is effective in visualizing tumor angiogenesis and hypoxia related to the malignancy of the cancerous lesion such as thyroid cancer and breast cancer[40–47]. In cardiovascular imaging, the ability of PAI to detect lipids has been exploited to characterize and monitor atherosclerotic plaques[48–51]. PAI can visualize vasculature and thus help to assess peripheral vascular diseases[21,52–55]. PAI can visualize microvascular structure and dynamics, particularly in the skin[56].
Sign up for Advanced Imaging TOC. Get the latest issue of Advanced Imaging delivered right to you!Sign up now
Among these clinical applications, PAI is especially useful for diagnosing and monitoring skin diseases, such as inflammations, wounds, and skin cancer, which are accompanied by abnormal changes in the vasculature or melanin distribution[57–59]. For example, melanoma, psoriasis, dermatitis, and port-wine stains (PWS) have distinct structures of blood vessels around lesions, and melasma and melanoma are strongly associated with abnormal melanin distribution[60–66].
Conventional diagnostic tools for skin diseases include skin biopsies and optical or US imaging, but these methods are invasive, require subjective evaluation, or have low sensitivity. Skin biopsy for histological examination is the gold standard for diagnosing many skin diseases, but it leaves a wound and can facilitate infection; it is also impractical for assessing large lesions[67]. Optical imaging such as photography or fluorescence imaging (i.e., Wood’s lamp) can be performed in vivo to visually evaluate the color, size, or boundary of the lesions, but it can only assess superficial skin features to depths of
In this review, we discuss clinical PAI techniques for imaging human skin to resolutions ranging from micrometers to millimeters. First, we introduce the principles of the PAI modalities used for skin imaging in three categories (Fig. 1) according to the scale: PA microscopy (PAM), PA mesoscopy (PAMes), and PA tomography (PAT). Next, we discuss PAI applications to image two types of imaging targets in the skin: vasculature only or vasculature plus melanin. To the best of our knowledge, there were only several recent PAI reports that discussed melanin imaging only, and thus we partially included the vascular imaging in the latter section. Each section is divided into three subsections that present uses of PAM, PAMes, and PAT, respectively, to compare their different imaging scales of the same imaging target. We review peer-reviewed papers published from 2018 to 2024, with a focus on imaging techniques for human skin, and on clinical indicators that consider vasculature and melanin. We then analyze the general relationship between spatial resolution and imaging depth or field-of-view (FOV) to guide the selection of an appropriate PAI modality for specific skin-imaging applications.
Figure 1.PAI modalities in skin imaging. (a) A schematic of the PAI principle (left) and the optical absorption spectra of
2. Clinical PAI Modalities for Skin Imaging
The major components of PAI systems are the optical excitation source (i.e., laser) and the acoustic detector (i.e., US transducer). Both the optical beam delivery and acoustic detection can be made either focused or unfocused (or diffuse), depending on the desired imaging depth and spatial resolution. In biological tissues, scattering is more than US waves, so each photon can ballistically travel up to only
A typical PAM system consists of a single-element focused US transducer, a laser source (usually emitting
OR-PAM uses a tightly focused laser beam to achieve high lateral resolution typically in the range of a few micrometers. Recent improvements in OR-PAM can achieve sub-micrometer resolution using a transparent US transducer to increase the numerical aperture (NA) of the optical configuration[9]. However, beyond the depth limit (
AR-PAM relies on acoustic focusing by the US transducer to achieve lateral resolution typically in tens of micrometers, which is determined by the US frequency of the transducer, NA, and the corresponding focal spot size. In principle, AR-PAM and PAMes both use a diffuse laser in relatively deep depths, but AR-PAM may use a quasi-focused laser when switchably combined with OR-PAM. In such a switchable OR/AR-PAM configuration, the acoustic focus position can be shifted to either
Both OR- and AR-PAM acquire time-resolved amplitude data (i.e., A-line data) for a single point per laser pulse. The axial resolution depends on the US frequency of the transducer. To obtain 2D or 3D images, multiple A-lines must be acquired by raster scanning using mechanical stages. PAM images are formed directly from the data acquired at the focal depth without additional reconstruction algorithms, but AR-PAM may use synthetic-aperture focusing techniques to improve lateral resolution at out-of-focus depths[70]. Moreover, image post-processing techniques such as motion correction or vascular signal enhancement techniques can be applied to additionally improve the PAM images[71,72]. Further, deep-learning algorithms can be used on PAM images for automatic microvessel segmentation or virtual staining[73,74].
However, conventional mechanical raster scanning is time-consuming and susceptible to motion artifacts, so it has limited clinical applications. Scanning speed can be increased using advanced optical or acoustic scanning systems such as Galvo scanners, micro-electromechanical system (MEMS) scanners, or polygonal mirrors[75–79]. Laser scanning OR-PAM systems have been also proposed to increase imaging speed[80,81]. Instead of increasing scan speeds, some studies use deep learning to increase temporal resolution by achieving sufficient resolution while requiring less data than conventional methods[82]. Alternatively, the spatial light modulation technique enables high frame rate PAM imaging without mechanical scanning[83].
PAMes operates on a scale between AR-PAM and PAT, typically using wide-field diffuse laser illumination and a single-element focused US transducer. PAM is similar to AR-PAM in that it is an acoustic-resolution modality using a focused US transducer. However, AR-PAM is often configured with an optical lens to focus the laser to be switchable with OR-PAM. On the contrary, PAMes uses a diffused laser to deliver higher laser power to image deeper into the tissue. PAMes stacks multiple A-lines to create 2D or 3D images, but it also uses reconstruction algorithms to increase spatial resolution and SNR[84]. The method usually achieves imaging depths of up to 10 mm with spatial resolutions in tens of micrometers. Particularly, raster-scan optoacoustic mesoscopy (RSOM) from iThera Medical GmbH, the most widely used PAMes system, uses ultra-broadband (
PAT targets the visualization of tissue structures within several centimeters in all directions. It uses wide-field laser illumination and a US transducer array (typically 64 to 256 elements) for tomographic image reconstruction and, therefore, can acquire a cross-sectional image (i.e., B-mode) with a single laser pulse[86]. For 3D imaging, PAT can either use a 1D transducer array (e.g., linear and curved) with a motorized scanner or a 2D transducer array that acquires volume data at once[87–89]. In addition, 3D handheld PAT systems have been developed for versatile imaging of healthy or diseased tissues positioned in narrow locations where conventional 3D PAI systems cannot fit in due to size[90,91].
PAT usually uses a low-frequency (several megahertz) US array transducer, which facilitates visualization of deeper tissues than PAM or PAMes at the cost of a loss of spatial resolution (to hundreds of micrometers). Furthermore, most US transducer arrays have a limited signal reception aperture and may not fully acquire the PA waves that propagate omni-directionally, so limited-view artifacts may develop. Therefore, advancements in image reconstruction are needed to maximize the achievable image quality even in the limited signal reception environment[92]. Recent studies have used deep learning to solve the low spatial resolution or limited-view artifacts of PAT systems[93,94].
PAT uses a wide-field laser and, therefore, does not rely on optical focusing and does not require a very high-PRF laser, as PAM does. Therefore, multiple optical wavelengths can be used relatively easily to estimate the relative concentrations of chromophores (e.g.,
We now compare the three PAI modalities described above (Fig. 1). The basic principle of PAI is that the laser is illuminated on the target tissue and the corresponding PA signal is generated according to the optical absorption coefficients of chromophores [Fig. 1(a)]. Typically, PAM and PAMes use the visible range to achieve high spatial resolution and sensitivity, whereas PAT can also use NIR when deep penetration is required. Many pulsed laser sources that use an optical parametric oscillator (OPO) support fast wavelength switching in the NIR range; this ability is suitable for multi-wavelength PAT or even some PAMes implementations.
The systems that use each modality have different constructions and applications, and they provide different types of images [Fig. 1(b)]. PAM and PAMes systems commonly use a focused US transducer with motorized raster scanners to acquire images, whereas PAT systems use unfocused US transducer arrays. In PAM, particularly for OR-PAM, the laser is focused at the epidermal depth (
3. Clinical PAI in Skin Vasculature Imaging
3.1. Clinical PAM Techniques for Imaging Skin Vasculature
Cheng et al. developed a typical OR-PAM system that can achieve centimeter-scale scan range (up to
Ahn et al. have combined OR-PAM and photoplethysmography (PPG) to track the movement of single blood microvessels and PPG signals simultaneously in a finger [Fig. 2(a)][96]. This implementation of OR-PAM used a galvanometer scanner, a flat ring-shaped US transducer, and a parabolic mirror. The galvanometer scanner allows fast B-mode scanning at a frame rate of 50 Hz, and the parabolic mirror steers both light and acoustic waves simultaneously with a short focal length and 2.5 µm lateral resolution. This system could simultaneously acquire cross-sectional vascular images and measure the blood volume of the finger, which were synchronous to the co-acquired PPG signals. The heart rates of three volunteers were measured using both OR-PAM and PPG, and the measurements by the two modalities agreed well.
Figure 2.PAM techniques for improved visualization of skin structures. (a) An OR-PAM system integrated with a photoplethysmography (PPG) sensor. Simultaneous real-time monitoring of PA and PPG signals from superficial dermal vessels in fingers accurately estimated the heartbeat rate (
Wang et al. have constructed a dual-modal PA/US dermoscope using a bimorph US transducer that uses lead zirconate titanate (PZT) (for US transmission) and polyvinylidene fluoride (PVDF) (for PA and US reception) [Fig. 2(b)][97]. PZT provided strong US wave generation, and the PVDF had wide signal reception bandwidth to provide high spatial resolution. The system achieved an axial resolution of 57.8 µm for both modalities, with a lateral resolution of 45 µm for US microscopy and 6.8 µm for PAM. A human palm could be imaged to a depth of
To increase the visibility and quantitative accuracy of PA dermoscopic images, a 4D spectral-spatial computational model of the skin has been developed[98]. It considers the heterogeneous optical and acoustic tissue parameters. The model was built with a Monte-Carlo simulation of optical propagation, k-Wave MATLAB toolbox for acoustic simulation, and detailed modeling of the multilayered skin structure in the 3D spatial domain as well as the spectral domain (i.e., optical wavelength). A computational skin model was used to generate in silico PA dermoscopy data pairs (low center frequency vs. high center frequency and strong vs. weak scattering) to train two U-Net neural networks to increase the spatial resolution and imaging depth. The trained network significantly increased the axial resolution and visibility of deep vessels in images of human palm skin.
Cheng et al. used an extended depth-of-field PAM (E-DOF-PAM) to monitor the revascularization process during the healing of a skin wound[99]. An elongated focus lens was added to a conventional Gaussian beam PAM (GB-PAM) system to create Bessel beams that allowed relatively uniform spatial resolution at different depths. Compared to GB-PAM, the lateral resolution of the E-DOF-PAM was slightly degraded from 4.7 to 6.5 µm, but the depth-of-field was significantly increased to nearly 1 mm. Wounded skin on a volunteer was imaged using E-DOF-PAM, and the image separately displayed the SC, stratum basale (SB), and dermal vascular layer. PAM images of the wound showed increased thickness and PA amplitude in the SC layer, disappearance of signals in the SB layer, and development of small and dense microvasculature in the dermal layer.
Ma et al. developed a non-invasive photoacoustic microscopic biopsy (PAMB) system to visualize and quantify dermal angiopathy with high spatial resolution and penetration depth[100]. The PAMB system integrates a 532 nm laser and a broadband (5–90 MHz) US transducer, and achieves axial resolutions from 34 to 57 µm and lateral resolutions from 1.5 to 104 µm (1.5 to 3.8 µm on the zero plane, 10 to 40 µm at 0.2 to 1.5 mm depth, and 40 to 140 µm at 1.5 to 3.0 mm depth in skin tissue), with a maximum imaging depth of 3 mm. This system uses an adjustable confocal opto-sono objective that allows switching among different NAs to optimize focus at various depths. The system quantified vascular parameters, including capillary-loop depth, diameter, and vascular volume, and it could distinguish healthy skin from PWS-affected skin (Fig. 3). PAMB demonstrated significant differences in vascular features among different PWS stages and, thereby, provided a quantitative assessment of lesion severity. The authors introduced a comprehensive parameter (PAFIND) that combines microvessel density and depth to enable objective evaluation of PWS severity and treatment efficacy. The study highlighted PAMB’s potential for non-invasive, label-free biopsies, and it may be a valuable tool for diagnosing and monitoring skin diseases and treatment responses.
Figure 3.Photoacoustic microscopic biopsy (PAMB) technique for port wine stain (PWS) lesion imaging. Compared to normal skins, PWS skins have dilated vascular structure in the dermal layer. MAP, maximum amplitude projection; EP, epidermis; DR, dermis; SB, stratum basale; SC, stratum corneum. The figures were reproduced with permission from Ref. [
3.2. Clinical PAMes Techniques for Imaging Skin Vasculature
Hindelang et al. used RSOM to differentiate between allergic and irritant skin reactions in patch testing[101]. The RSOM images were analyzed to provide quantified vascular biomarkers such as blood volume, vessel fragmentation, and frequency-content ratio. Allergic reactions showed higher vessel fragmentation and lower frequency ratios than did irritant reactions. The RSOM system used in this study consisted of a 532 nm laser and a 55 MHz US transducer. The US transducer has an ultra-broadband bandwidth of 10 to 120 MHz, so the RSOM provided images that had spatial resolutions of 8 µm axially and 30 µm laterally, and an imaging depth of 1.5 mm. The researchers divided the detected PA signal into two frequency bands, i.e., 10 to 40 MHz to distinguish large vasculature, and 40 to 120 MHz to distinguish small vasculature [Fig. 4(a)]. Compared to dynamic optical coherence tomography (D-OCT), RSOM provided similar image quality in en face images but better quality in transverse cross-sectional images due to its axial resolution and penetration depth.
Figure 4.(a) Cross-sectional RSOM image composed of two bandwidth signal images and a binary image of the high-frequency signal within ROI. The combined image of high-frequency signal (green, representing small vessels) and low-frequency signal (red, representing large vessels) shows the boundary between epidermis and dermis. The binary image is used to calculate the vessel fragmentation value. (b) The process of skin biomarker quantification in cross-sectional RSOM images. First, the EP and DR regions are divided, and the EP thickness and the EP signal density are calculated. Then, the vessel numbers are calculated by counting the vessel branches (red dots) in the DR area, and the total blood volume is calculated from the binary image. RSOM, raster scan optoacoustic mesoscopy; ROI, region of interest; EP, epidermis; DR, dermis; VS, vessel segmentation; BC, biomarker computation. The figures were reproduced with permission from Refs. [
The RSOM technique has also been used to quantify skin sensitivity to UV radiation in healthy volunteers[102]. Skin areas were exposed to increasing doses of UV radiation, and the dermal microvasculature was monitored in RSOM images. UV radiation induced vasodilation and recruitment of vessels, and the vessel fraction increased linearly with UV doses. These results suggest that RSOM can monitor and quantify UV-induced erythema with high precision.
Nau et al. used RSOM to measure biomarkers such as the mean capillary loop length and mean plexus width to assess the severity of atopic dermatitis (AD)[103]. Yew et al. also used the method to investigate skin structure and vasculature changes in AD lesions during treatment[104].
Li et al. used multispectral raster scanning optoacoustic mesoscopy (ms-RSOM) with four distinct wavelengths (532, 555, 579, and 606 nm) to obtain functional information of AD lesions[105].
Vascular indicators in the skin provided from RSOM images have also been used to assess diabetes-related changes in dermal microvasculature [Fig. 4(b)][107]. RSOM images of diabetes patients were compared to those of healthy volunteers; they were segmented and compared to derive six label-free PA biomarkers: epidermal thickness, signal density of epidermis layer, dermal blood volume, vessel diameter, small vessel number, and large vessel number. Microvascular parameters differed between diabetic patients and healthy people, with the difference being most significant in the number of vessels
However, the RSOM technique has the disadvantage of a long scan time. To solve this problem, He et al. developed a fast RSOM (FRSOM); it significantly reduces scanning time and, thereby, minimizes motion artifacts[108]. The FRSOM system consists of a coaxial optical-acoustic probe that uses a 532 nm laser and a 50 MHz spherically focused ring transducer with an integrated pre-amplifier to increase the SNR. Thus, FRSOM could obtain the same image quality as conventional RSOM with lower laser-pulse energy; this trait allowed an increased laser repetition rate without exceeding the laser safety limit, so scanning can be accelerated. To scan a
3.3. Clinical PAT Techniques for Imaging Skin Vasculature
Systemic sclerosis (SSc) is an immune-mediated disease that involves fibrosis and vasculopathy of the skin and internal organs. SSc has a higher mortality rate than any other rheumatic disease[109]. PAI has been evaluated as a method to detect changes in skin microvessels to enable early detection and quantitative assessment of these conditions. Masthoff et al. used MSOT Acuity Echo (iThera Medical, Munich) to analyze fingers that were afflicted with SSc[110]. Each cross-sectional image was
Figure 5.PAT techniques for clinical skin vasculature imaging. (a) MSOT principle and image processing step. (b) MSOT images of HbR,
RH is a non-invasive method to assess peripheral microvasculature, which, in the simplest form, represents the magnitude of limb reperfusion following a brief period of ischemia induced by arterial occlusion[111]. Using MSOT imaging, Rodrigues et al. showed that PAI can be used to observe and quantify RH in vivo[112]. Superficial and deep vascular plexuses were measured at baseline, during occlusion, and after occlusion, and the corresponding HbR,
Zhang et al. used a PAI system that uses LEDs (AcousticX, CYBERDYNE, Inc., Tsukuba, Japan) to analyze the severity and treatment response of PWS[113]. The system consists of a US transducer array with 128 elements and 9 MHz center frequency, and two LED arrays that had 400 µJ pulse energy, one attached on each side of the US transducer. The system provided mean axial resolution of 220 µm and mean lateral resolution of 460 µm, with an imaging depth of up to 28 mm. PWS legions were imaged during treatment using hematoporphyrin monomethyl ether photodynamic therapy. After treatment, PWS levels estimated from the PA signal intensity showed significant decrease.
4. Clinical PAI in Skin Vasculature and Pigmentation Imaging
4.1. Clinical PAM Techniques for Imaging Skin Vasculature and Pigmentation
Ma et al. reported a switchable OR-AR PAM system to image the melanin and vasculature in different skin layers of human skin [Fig. 6(a)][114]. A customized adjustable opto-sono objective lens was used to adjust the focal position in the axial direction, and it enabled switching between OR and AR modes. For instance, OR imaging was used when the opto-sono objective lens was positioned with its optical focus of
Figure 6.Switchable OR-AR PAM techniques for skin vasculature and pigment imaging. (a) Switchable OR-AR PAM technique that alters the focusing mode by axially translating the depth of the fixed focus. (b1)–(b3) Autofocusing PAM technique using a voltage-controlled liquid lens. (b1) The focal sizes of the optical and acoustic beams were controlled by the input voltage at the electrodes. (b2) Phantom imaging demonstrated that autofocusing improved the imaging depth compared to the fixed focus mode. (b3) A healthy volunteer’s palm skin was imaged in different layers, where SC/SB layers and Va layers contained the melanin and vasculature, respectively. OR, optical resolution; AR, acoustic resolution; EP, epidermis; DP, dermal papillae; RD, reticular dermis; Hy, hypodermis; SC, stratum corneum; SB, stratum basale; SVP, superficial vascular plexus; DVP, deep vascular plexus; E-D, epidermis-dermis junction; VN, vascular network; PVDF, polyvinylidene fluoride. The figures were reproduced with permission from Refs. [
Instead of scanning with a fixed focal depth, an autofocusing opto-sono probe has been developed for dynamic confocusing along the skin contour [Figs. 6(b1)–6(b3)][115]. The main working component of the autofocusing probe was a liquid lens that exploits electrowetting; it consists of 22% NaCl solution as the conducting liquid and a mixture of glycerol and silicone oil as the insulating liquid. When electrical voltage was applied between the adjacent electrodes, the curvature radius of the interface between the two liquids was altered, so the optical and acoustic beams were refracted simultaneously to the same angle. Using real-time feedback of PA A-line signals, the autofocusing probe adjusted the focus to maximize the PA amplitude and, thereby, increased the SNR at deeper depths compared to fixed-focus scanning.
Ma et al. investigated the use of OR-PAM to measure the melanin component of café-au-lait macules (CALMs) in patients[116]. The researchers developed a fast linear confocal scanning PA dermoscopy (FLCS-PAD) system, in which a dual PVDF transducer created a laterally elongated acoustic focal field (
A multiscale confocal PA dermoscopy (MC-PAD) system has been developed using multiple objectives (e.g.,
Wang et al. introduced bifocal dual-wavelength (532 and 1064 nm) PAM to improve the visualization of deep dermal vessels in PWS patients (Fig. 7)[118]. The conventional 532 nm wavelength could visualize the epidermis and superficial vascular plexus, and the additional 1064 nm wavelength enabled tissue visualization up to
Figure 7.Bifocal dual-wavelength PAM technique for port wine stain (PWS) characterization. (a) Two optical wavelengths, 532 and 1064 nm, were focused at different depths and superposed to provide elongated focal zone and deep imaging depth up to 3 mm. The epidermis (EP) and superficial vascular plexus (SVP) were imaged at 532 nm and deep vascular plexus (DVP) at 1064 nm. (b) Compared to normal skin, PWS skin expressed less melanin in the EP layer and more vasculature in the SVP and DVP layers. DE, dermis; Hy, hypodermis; SC, stratum corneum; ED, epidermal-dermal junction; PWS, port wine stain; PA, photoacoustic; and a.u., arbitrary unit. The figures were reproduced with permission from Ref. [
A commercially available PAM system (PASONO-SKIN, Guangdong Photoacoustic Technology Co., Ltd., Foshan, China) has been used to classify melasma by quantifying the clinical indicators for melanin and blood vessels[119]. The PASONO-SKIN system consists of a 532 nm nanosecond pulsed laser and a ring US transducer (center frequency: 35 MHz, bandwidth: 114%, focal length: 8 mm) and achieves a resolution of 50 µm axially and 7 µm laterally. The conventional classification of melasma has been complex due to various mixtures of pigment abnormalities and vascular formation. In this aspect, PAM measurements on the depth of melanin, mean vascular diameter, and vascular density were used to classify the melasma lesions quantitatively. The depth of melanin determined whether the lesion is the epidermal type (melanin only in the epidermis) or mixed (melanin in both epidermis and dermis), and the two vascular parameters further classified the lesions into M + V (i.e., increased vasculature) or M types (i.e., non-significant increase in the vasculature). PAM provided 3D structure data of melanin and blood vessels in the epidermis and dermis [Fig. 8(a)], and the images were quantified into the PA amplitude (arbitrary units, a.u.) of melanin and mean diameter (μm) and density (%) of vessels. As classified by the metrics, all types of lesions had a significant increase in the PA amplitude of melanin, but only the M + V type showed a significant increase in the vascular diameter and density [Fig. 8(b)]. The results showed that PAM could provide quantitative parameters for classifying melasma type.
Figure 8.Classification of melasma lesion types using PAM. (a) The PAM images are from a patient with epidermal M + V type melasma, showing denser melanin in the epidermal layer (
4.2. Clinical PAMes Techniques for Imaging Skin Vasculature and Pigmentation
He et al. showed that FRSOM can distinguish melanoma lesions from benign nevi by quantifying differences in vascular density, complexity, and tortuosity between the two types of lesions[120]. FRSOM images (Fig. 9) distinguish small vasculature from large vasculature structures by reconstructing data from two frequency bands: high frequency (40–120 MHz, Fig. 9, green) and low frequency (10–40 MHz, Fig. 9, red). The FRSOM system showed that an irregular dermal vasculature appears in the tumor area and is resolved in the edge of the lesion. However, to separate the effects of the melanin signal, the boundaries must be segmented. Therefore, the authors implemented dual-wavelength (515 and 532 nm) FRSOM to separate the melanin and Hb using spectral unmixing. The dual-wavelength FRSOM took about 25 s to scan an area of
Figure 9.RSOM images of a melanoma lesion and the surrounding skin tissue. (a) A photograph of a melanoma lesion. Arrows indicate the three scanning positions. The red rectangle depicts the FOV of
When the goal is to distinguish signals from multiple chromophores, a multi-wavelength laser must be used. Berezhnoi et al. developed ultrawide spectrum and bandwidth raster scan optoacoustic mesoscopy (UWSB-RSOM) to visualize the biomolecules in human skin using a laser that has a broad spectral range from visible to short-wave infrared (SWIR) [Fig. 10(a)][121]. The UWSB-RSOM system used an Nd:YAG OPO laser to generate
Figure 10.UWSB-RSOM system configuration and cross-sectional USWB-RSOM images over 5 wavelengths. (a) Schematic of UWSB-RSOM and a structure of the imaging target (skin). (b1)–(b6) Cross-sectional RSOM images of skin over 5 wavelengths. (b1), (b2) Distribution of hemoglobin and melanin in the skin at 515 and 532 nm. Arrows indicate the position of hair. (b3) Melanin in the epidermis and hair at 650 nm. (b4) Subcutaneous fat, sebaceous glands (marked with arrows 1 and 2), and water content in the epidermis at 1210 nm; arrow 3, sebum content on the hair shaft. (b5) Water distributed in the epidermis at 1450 nm. (b6) Composite of images at all five wavelengths. ADC, analog-to-digital converter; AMP, 60 dBm amplifier; CL, collimating lens; D, dermis; EP, epidermis; FB, fiber bundle; FL, focusing lens; H, UWSB-RSOM holder; HPF, high-pass filter; HS, hair shaft; HB, hair bulb; IU, detachable interface unit; M, mirror; PBS, polarizing beam splitter; S, schematic of human skin; SF, subcutaneous fat; SG, sebaceous glands; UT, ultrasound transducer. The figures were reproduced with permission from Ref. [
4.3. Clinical PAT Techniques for Imaging Skin Vasculature and Pigmentation
Much PAT research to image joint vasculature and pigment has targeted diagnosis of melanoma, which is a malignant tumor caused by the transformation of melanocytes, and that accounts for most deaths from skin cancer. A person with melanoma is most likely to survive when diagnosis is early and treatment is performed before the melanoma metastasizes. To identify the progression stage (according to the Breslow depth), the size and thickness of melanoma lesions must be quantified, and lesions must be treated or excised. Melanoma lesions contain high concentrations of melanin that produce a significantly stronger PA signal against normal skin and, therefore, can be distinguished from surrounding tissue.
Breathnach et al. used a commercial Vevo LAZR (VisualSonics Inc., Toronto, Canada) system with LZ 550 probe (VisualSonics Inc., Toronto, Canada) to scan melanomas[122]. The system includes a 256-element linear array transducer with a high center frequency of 40 MHz. A tunable OPO laser (680–970 nm, OPOTEK, Carlsbad, USA) can be used with a fiber bundle to irradiate the laser from both sides of the transducer array at 30° to the imaging plane.
Figure 11.PAT techniques for melanoma imaging. (a) Clinical photograph of a melanoma. (b) 3D PA scan image of the black dashed region in (a). (c) Co-registration of PA and B-mode US images of
Kim et al. imaged an excised melanoma skin sample using a clinical PA/US imaging system that combines a clinically viable research US system (ECUBE-12 R, Alpinion Medical Systems, Anyang, Korea) and an OPO laser (Phocus Mobile, OPOTEK, Carlsbad, USA) to generate three wavelengths (680, 800, and 1064 nm)[124]. To image the whole lesion, a linear motorized scanning system moved the imaging probe along the elevation direction and then stacked cross-sectional PAT images. The spatial resolutions were 0.2 mm axially and 1.2 mm laterally, and the scanning range was
PAT has also been evaluated for diagnosis of non-melanoma skin cancers (NMSCs), which constitute most skin cancers. The lesions infiltrate skin tissue to uneven depths, and their boundaries can be difficult to identify, so these carcinomas are treated using Mohs micrographic surgery, which performs repeated excision and examination. This consumes much time, so the boundary and the size of the lesion must be estimated accurately before the surgery.
Kukk et al. modified their integrated OCT-Raman spectroscopy device to perform PA imaging. The modified system uses a single-crystal US transducer (L22-14vX-LF, Vermon, France) that includes 128 elements with a central frequency of 18 MHz[126]. An OPO laser (SpitLight Compact 400 OPO, InnoLas Laser GmbH, Germany) emits maximal pulse energy at 430 nm wavelength with 7 ns pulse duration and 20 Hz repetition. This system had resolutions of 200 µm axially and 100 µm laterally for US, and 300 µm axially and 200 µm laterally for PA, with a PA imaging depth of up to 5 mm. In vivo tests on human skin nevi demonstrated a good correlation between PA measurements and histological results [Fig. 12(a)], although tissue shrinkage or position-matching errors caused differences between the thickness measurements by histology and by PAI. The authors also imaged skin lesions that were suspected to indicate skin cancers[127]. PA/US image measurements of lesion thickness correlated strongly (
Figure 12.PAT techniques for skin lesions. (a) Comparison of combined
Attia et al. imaged skin lesions suspected to be non-melanoma carcinomas using an inVision 512-echo MSOT system (iThera Medical GmbH, Munich, Germany)[128]. The FOV of the system was
PAI can also be used to differentiate between benign and malignant skin tumors, by exploiting its ability to distinguish between blood vessels and melanin. For instance, skin tags are commonly benign skin lesions, but they can be malignant lesions like squamous cell carcinoma, BCC, or melanoma. Therefore, a sensitive diagnostic modality is needed to distinguish the malignant lesions accurately. Fredman et al. evaluated reflectance confocal microscopy (RCM), OCT, and MSOT to analyze skin tags to provide lesion dimensions and micromorphological distributions of vascularization and melanin[130]. The MSOT imaging used MSOT Acuity (iThera Medical GmbH, Munich, Germany), in which the US transducer array had 256 elements with 8 MHz central frequency and optical wavelengths of 700, 730, 760, 780, 800, 850, and 875 nm. The FOV was
5. Discussion
In the papers reviewed here, both imaging depth (Fig. 13, left) and lateral FOV (Fig. 13, right) show a trade-off relationship with spatial resolution. Least squares line fitting showed an
Figure 13.Specification plots relating the spatial resolution, imaging depth, and lateral FOV of clinical PA skin imaging systems. The overall relationship of the imaging depth versus spatial resolution (left) and the lateral FOV versus spatial resolution (right) is presented. Dotted lines show reference tradeoff lines with a given ratio, and the blue dotted line is obtained from least-square fitting including both axial (triangle marker) and lateral (circle marker) resolutions. PAM, photoacoustic microscopy; PAMes, photoacoustic mesoscopy; PAT, photoacoustic tomography; FOV, field of view; PA, photoacoustic. (Refs. PAM- [
Systems for skin imaging have a range of specifications PAM (Table 1), PAMes (Table 2), and PAT (Table 3). Generally, PAM and PAMes offer much higher axial/lateral resolutions (
Ref. | Modality | Laser wavelength (nm) | US transducer | US frequency | FOV, lateral × elevation × axial (mm × mm × mm) | Spatial resolution (μm) | Scan time |
[ | OR-PAM | 532 | Focused | Center: 40 MHz BW: 110% | Lateral: 5 Axial: 31.2 | ||
[ | OR-PAM + PPG | 532 | Flat ring transducer | Center: 15 MHz | Lateral: 1 Axial: 1 | Lateral: 2.5 Axial: 68.4 | 0.02 s per B-scan |
[ | OR-PAM + USM | 532 | Dual (coaxial annular, outer-PZT, inner-PVDF), focused | US excitation (PZT) Center: 33.4 MHz BW: 19.5–47.2 MHz PA/US reception (PVDF) Center: 35 MHz BW: 11.8–58.2 MHz | Lateral: | Lateral: 45 (US), 6.8–7.1 (PA) Axial: 57.8 (US) | NA |
[ | OR-PAM + Deep learning | 532 | Focused | Center: 20 MHz, BW: 100% | NA | NA | |
[ | Bessel-beam OR-PAM | 532 | Focused ring transducer | Center: 40 MHz BW: 120% | Lateral: 6.5 (1 mm DOF) Axial: 35 | NA | |
[ | OR/AR-PAM | 532 | Focused annular PVDF | Center: 42 MHz | Lateral: 10–40 (0.2–1.5 mm depth) Axial: 34 | 3–5 min per C-scan | |
[ | Switchable OR/AR-PAM | 532 | Focused | Center: 45 MHz BW: 7–83 MHz | Lateral: 4.4 (OR), 47 (AR) Axial: 35 | 0.5 s per B-scan, | |
[ | Switchable OR/AR-PAM | 532 | Autofocusing PVDF-based transducer | Center: 32.8 MHz BW: 45.4 MHz | Lateral: 9.8 (OR, variable with the applied voltage) Axial: | NA | |
[ | OR-PAM | 532 | Focused PVDF | BW: 10–75 MHz | Lateral: 4.2 Axial: 41 | ||
[ | OR/AR-PAM | 532 | Focused annular PVDF | Center: 45 MHz BW: 5–85 MHz | Lateral: 3.8, 2.3, 1.5 (for | NA | |
[ | Dual-wavelength bifocal OR-PAM | 532, 1064 | Focused ring transducer | Center: 30 MHz | Lateral: | Lateral: 6.6 (532 nm), 46.8 (1064 nm) Axial: 50.6 | NA |
[ | Commercially available PAM (PASONO-SKIN) | 532 | Ring transducer | Center: 35 MHz BW: 114% (15–55 MHz) | Lateral: 7 Axial: 50 | NA |
Table 1. Specifications of PAM Systems for Clinical Skin Imaging.
Ref. | Modality | Laser wavelength (nm) | US transducer | US frequency (MHz) | FOV, lateral × elevation × axial (mm × mm × mm) | Spatial resolution (µm) | Scan time |
[ | RSOM | 532 | Focused transducer | Center: 55 BW: 10–120 | Lateral: 30Axial: 8 | 70 s per C-scan | |
[ | RSOM | 532 | Focused transducer | Center: 55 BW: 10–120 | Lateral: 30, Axial: 7 | 80 s per C-scan | |
[ | RSOM | 532 | Focused transducer | Center: 55 BW: 10–120 | Lateral: 30, Axial: 8 | 70 s per C-scan | |
[ | RSOM | 532 | Focused transducer | Center: 55 BW: 10–120 | Lateral: 30 Axial: 8 | 70 s per C-scan | |
[ | FP-RSOM | 532 | Focused hole transducer | Center: 50 BW: 10–120 | Lateral: 30 Axial: 7 (same as RSOM) | ||
[ | FRSOM | 532, 555, 579, and 606 | Focused hole transducer | Center: 50 BW: 10–120 | Lateral: 30 Axial: 7 (same as RSOM) | 15 s (single) 60 s (multi) per C-scan | |
[ | UWSB-RSOM | 650, 1210, 1450, 515, and 532 | Two spherically focused piezoelectric transducers | Center: 23.9, 55 | Lateral: 4 Axial: 2 | At 23.9 MHzLateral: 53 MHzAxial: 33 At 55 MHz Lateral: 29.6 Axial: 8.6 |
Table 2. Specifications of PAMes Systems for Clinical Skin Imaging.
Ref. | Modality | Laser wavelength (nm) | US transducer | US frequency | FOV, lateral × elevation × axial (mm × mm × mm) | Spatial resolution (µm) | Scan time |
[ | MSOT | 700 to 850 | Linear array transducer | Center: 4 MHz BW: 52% | Lateral: 30 mm Axial: 30 mm | NA | |
[ | MSOT | NA | NA | NA | NA | NA | |
[ | LED based PAI | 850 | Linear array transducer | Center: 9 MHz | Axial: 38 mm | Lateral: 460 | 20 s per B scan 1–3 min per C-scan |
[ | PAISU | 680, 700, 750, 850, and 900 | Linear array transducer | Center: 40 MHz BW: 55% | NA | NA | |
[ | PA/US | 700, 756, 796, 866, and 900 | Linear array transducer | Center: 8.5 MHz BW: 3–12 MHz | Lateral: 1000 Axial: 200 | ||
[ | PA/US | 430 | Single crystal transducer | Center: 18 MHz BW: 67% | Axial: 5 | Lateral: 300 Axial: 200 | |
[ | PA/US | Single: 430 or 530 | Single crystal transducer | Center: 18 MHz BW: 67% | Axial: 5 | Lateral: 300 Axial: 200 | |
[ | MSOT | NA | NA | NA | NA | NA | NA |
[ | vMSOT | 700, 715, 730, 760, 780, 790, 800, 825, 850, and 900 | Spherical matrix array transducer | NA | 80 (isotropic) | ||
[ | OCT, RCM, and MSOT | 700, 730, 760, 780, 800, 850, and 875 | Hemispherical array transducer | Center: 8 MHz BW: 60% | NA | ||
[ | HFUS, RCM, OCT, and PAI | NA | NA | NA | NA | NA |
Table 3. Specifications of PAT Systems for Clinical Skin Imaging.
AR-PAM techniques can be implemented with almost the same specifications as PAMes because they are both built on a US focus. However, PAMes systems, often known as RSOM, offer a fine axial resolution of
Especially for PAM, the lateral resolution coarsens as the optical wavelength increases, so
PAT has much coarser spatial resolution PAM or PAMes methods, but it allows large transverse scan areas (
The three PAI modalities use different analysis metrics related to skin vasculature (Table 4) or vasculature plus pigment structures (Table 5). The differences in the diagnostic information can be summarized as follows: PAM and PAMes focus on analyzing the detailed micro-scale vascular or melanin structures layer by layer, whereas PAT focuses on measuring the dimensions of a whole lesion or relative concentrations of Hb or melanin. In skin diseases including allergies and atopy, accompanying superficial changes such as inflammatory reactions, PAM or PAMes could be useful for detecting changes in skin thickness and vascular structure and determining the effectiveness of treatments. They can also be used in other skin pigment disorders such as PWS to analyze and quantify the characteristic features of pigment and vasculature. For skin diseases with macroscopic lesions such as melanoma, it is important to assess a whole lesion dimension and determine whether a lesion is benign or not. In this sense, PAT can provide a full scan of an entire lesion for a holistic view of melanin and vessel distribution changes reaching the deep subcutaneous layer under the skin. It is relatively easy to apply multi-wavelength imaging, which can be used to specifically differentiate between melanin and vascular signals. The different analytic roles of PAI modalities are most apparent when looking at studies on target disease common to all three modalities. For instance, in PWS imaging studies, PAM had the advantage of imaging capillaries in a small FOV and can quantify the density and depth of blood vessels in lesions. Using the microstructural information, PAM can also provide quantitative diagnostic indices that are sensitive to alterations in the shallow skin layers. PAT can cover large skin areas that include almost an entire PWS lesion, whereas PAM can only analyze a small sample area of a lesion. PAT has poor spatial resolution and, therefore, cannot identify and analyze vascular geometry, but it can distinguish lesions by considering their relative signal intensities and indexing their severity.
Ref. | Imaging target | Analysis metrics | Key features & findings |
[ | Volunteer’s opisthenar and palm areas | Visual assessment of the number of capillaries, depth distribution, and vessel diameter | Imaging depth was greater in the palm than in the opisthenar due to higher melanin concentration in the epidermis |
[ | Volunteer’s finger | Vascular movements and changes in the blood volume | The vascular movements and the heartbeat rates measured with both OR-PAM and PPG well matched between the two modalities |
[ | Volunteer’s palm | PA: Epidermal (0–90 µm), epidermal-dermal junction (90–210 µm), dermis (210–350 µm), and vessel plexus (350–1500 µm). US: big vessel (1.5–2.6 mm) and fibrous tissue (2.6–5 mm) | The PA and US microscopic images characterized the microvasculature information and full structures of skin in vivo, respectively |
[ | Volunteer’s palm | Axial resolution and deep vessel visibility | Deep learning model improved the epidermal layer differentiation and visibility of deep vessels |
[ | Traumatized skin | SC layer thickness and PA amplitude, SB layer PA amplitude, and dermal vessel diameter | Increased thickness and PA amplitude in the SC layer, disappearance of signals in the SB layer, and development of small and dense microvasculature in the dermal layer |
[ | PWSpre- and post-PDT treatment | Density, depth, and diameter of dermal vessels | Density, depth, and diameter of lesion vessels were reduced after PDT where the effects were more significant in superficial dermis than in deep dermis |
[ | Allergic and irritant skin reactions in patch testing | Blood volume, vessel fragmentation, and frequency content ratio | Allergic reactions showed higher vessel fragmentation and lower frequency ratios compared to irritant reactions |
[ | UV radiation region in volunteers’ skin | Vessel visibility and vessel diameter | RSOM imaging revealed UV-induced recruitment of previously non-perfused vessels and vasodilation, visible as an increase in vessel diameter |
[ | Patient with moderate atopic dermatitis | Epidermis thickness, total blood volume, and vessel diameter | 32% reduction in epidermis thickness, 10% decrease in total blood volume, and 26% reduction in vessel diameter post-treatment |
[ | Pretibial region of the participants with diabetes mellitus and age-matched healthy volunteers | Epidermal thickness, signal density of epidermis layer, dermal blood volume, vessel diameter, small vessel number, and large vessel number | The analysis revealed significant differences in microvascular parameters between healthy individuals and diabetes patients, with smaller vessels ( |
[ | Healthy individuals, smokers, and CVD patients | Vessel diameter, blood volume, and vessel density | Significant differences in microvascular ED biomarkers among healthy individuals, smokers, and CVD patients, providing a novel tool for early diagnosis and monitoring of cardiovascular risks |
[ | Fingers of patients with SSc and healthy volunteers | HbR, | Assessing microvascular dysfunction in SSc. Patients with progressive SSc had significantly lower MSOT values compared to patients with stable disease and healthy volunteers |
[ | Healthy ventral forearm with RH | PAT observations of the RH identified superficial and deeper vascular structures parallel to the skin surface as part of the human skin vascular plexus. PAT revealed that the suprasystolic occlusion impacts both plexus differently | |
[ | Patients with facial PWS | “PWS level,” indicating the severity of PWS lesions | The decrease in PWS levels after treatment based on PAI-association indicates better repeatability in assessing response to treatment compared to dermatologist-assigned clearance scores. It is a new quantitative assessment method for PWS severity and treatment response |
Table 4. PAI Analysis Features of the Skin Vasculature.
Ref. | Imaging target | Analysis metrics | Key features & findings |
[ | Volunteer’s opisthenar and palm areas | Melanin concentration of the SC (0–100 µm) and the SB (100–200 µm), vascular density below the SB (200–2000 µm) | Melanin concentration was significantly smaller, and vascular density was higher in the palm skin than in the opisthenar skin |
[ | CALM before and after Q-switched ruby laser treatment | PA amplitude of the SC and SB, epidermal thickness, and melanin concentration of CALM patient | Melanin concentration and epidermal thickness were significantly higher in the CALM area than in normal skin. Melanin concentration was significantly reduced after laser treatment |
[ | CALM, PWS | CALM: melanin concentration, SB thickness; PWS: microvessel density, vascularization increase | CALM skin had a higher PA signal intensity and thicker SB than that of healthy skin. Dermal vessels had greater diameters and were denser in appearance in PWS skin than in healthy skin |
[ | PWS | Melanin density in epidermal layer, vessel density and diameter in superficial dermal, and deep dermal layers | In PWS, the epidermal melanin density was lower, the superficial dermal vessels were significantly increased, deep dermal vessels had more branches, and vascular diameter in superficial dermis tended to be thicker |
[ | Melasma | PA amplitude and depth of epidermal melanin, mean dermal vascular diameter, and mean dermal vascular density | The PA amplitude of the epidermis, mean vascular diameter, and density in lesional skin were significantly higher than that in non-lesional skin. Mean vascular diameter and density were important in classifying melasma types M and M + V |
[ | 10 melanoma lesions and 10 benign nevi | Vascular density, complexity, and tortuosity | The FRSOM system effectively distinguished melanoma from benign nevi, providing valuable biomarkers for lesion characterization and improving diagnostic accuracy |
[ | Forearm skin of healthy volunteers | Fat, sebaceous glands, hair follicles, and microvasculature | UWB-RSOM distinguished between melanin and melanoidins in the epidermis, identified lipid distributions in the SWIR range, and visualized water content at 1450 nm |
[ | Patients with pigmented cutaneous lesions suspicious of melanoma | Tumor thickness | The photoacoustically measured lesion thickness gave a high correlation with the histological thickness measured from resected surgical samples |
[ | Melanoma patients | Tumor depth | The 3D multispectral photoacoustic imaging not only provides well-measured depth and sizes of various types of melanomas but also visualizes the metastatic type of melanoma |
[ | Patient with skin nevi | Maximal nevi depth | Developed a single-head co-localized US/PAT imaging system allowing for structural and depth measurement with the application for non-invasive diagnosis of skin cancer. The C-mode measured dermal structure and melanocytic depth correlated well with the histology |
[ | Patients who had suspicious skin lesions identified by dermatologists as potentially indicative of skin cancer | Thickness of the lesion at its thickest position | The results procured in our study underscore the potential of combined ultrasound and photoacoustic tomography as a promising non-invasive 3D imaging approach for evaluating human nevi and other skin lesions |
[ | Patients presented with lesions suspicious of non-melanoma carcinomas | Lesion dimensions | The dimensions were then correlated from the measurements acquired from histology, showing a good correlation via the intraclass correlation coefficient |
[ | Patients with lesions suspicious of non-melanoma skin cancer | Tumor depth and length | A statistically significant correlation for both tumor depth and length was found between vMSOT and histologic analysis |
[ | Patients with skin tags | MSOT supplemented with spatial distribution of melanin and | |
[ | Patients with pigmented skin lesions | OCT, RCM, and PAI in combination enable image-guided bedside evaluation of suspicious pigmented skin tumors |
Table 5. PAI Analysis Features of the Skin Vasculature and Pigmentation.
While reviewing the recent literature, we found that most of the clinical skin PAI research was based on vascular imaging, and not many weights were put on the melanin analysis. PAI is able to visualize the non-superficial morphology and distribution of skin pigment, and we think it is worth investigating its clinical potential to provide a critical triaging modality for various pigmentation diseases such as melanoma or hypopigmentation disorders. To expand the range of application, more skin biomarkers having optical contrast (e.g., collagen and lipid) should be discovered with the use of multi-wavelength laser sources supporting up to the NIR-II range.
In terms of clinical application, niche positioning in the current clinical flow would be important. Generally speaking, many skin diagnoses are made from qualitative visual inspection often aided by optical techniques (e.g., Wood’s lamp) or histologically analyzed by biopsy. In this aspect, PAI has great potential to fit between the two diagnostic methods, providing detailed visualization of non-superficial skin structures and quantitative analysis of the structural features. Still, the reliability and repeatability of PAI modalities should be validated in comparison to conventional techniques, and especially the effect of skin color on the PAI results should be investigated thoroughly. Due to the nature of optical and acoustic beams, the existence of thick scars, scabs, or calluses could also limit the application of PAI. Furthermore, PAI systems should be designed with extra care for hygiene because they require direct contact with the skin.
6. Conclusion
Overall, PAI technology can diagnose human skin non-invasively and potentially meet a variety of needs in dermatology such as staging, progression, and treatment monitoring. PAI also provides quantifiable metrics by analyzing the PA signal amplitudes that represent Hb or melanin contents observed in various scales using PAM, PAMes, or PAT. By adopting a fit-for-purpose PAI system, dermatologists will be able to effectively diagnose and test skin diseases, and reduce the number of repetitive treatments.
References
[1] A. G. Bell. The photophone. J. Franklin Inst., 110, 237(1880).
[3] M. Xu, L. V. Wang. Photoacoustic imaging in biomedicine. Rev. Sci. Instrum., 77, 041101(2006).
[33] S. Hu et al. Label-free photoacoustic ophthalmic angiography. Opt. Lett., 35, 1(2010).
[67] K. Abhishek, N. Khunger. Complications of skin biopsy. J. Cutan. Aesthet. Surg., 8, 239(2015).
[88] R. A. Kruger et al. Dedicated 3D photoacoustic breast imaging. Med. Phys., 40, 113301(2013).
[109] C. P. Denton, D. Khanna. Systemic sclerosis. Lancet, 390, 1685(2017).
[128] A. E. Attia et al. Non-invasive photoacoustic 3D imaging of non-melanoma skin cancers in Asian population, CF3B.7(2018).
[132] J. Yao, L. V. Wang. Sensitivity of photoacoustic microscopy. Photoacoustics, 2, 87(2014).
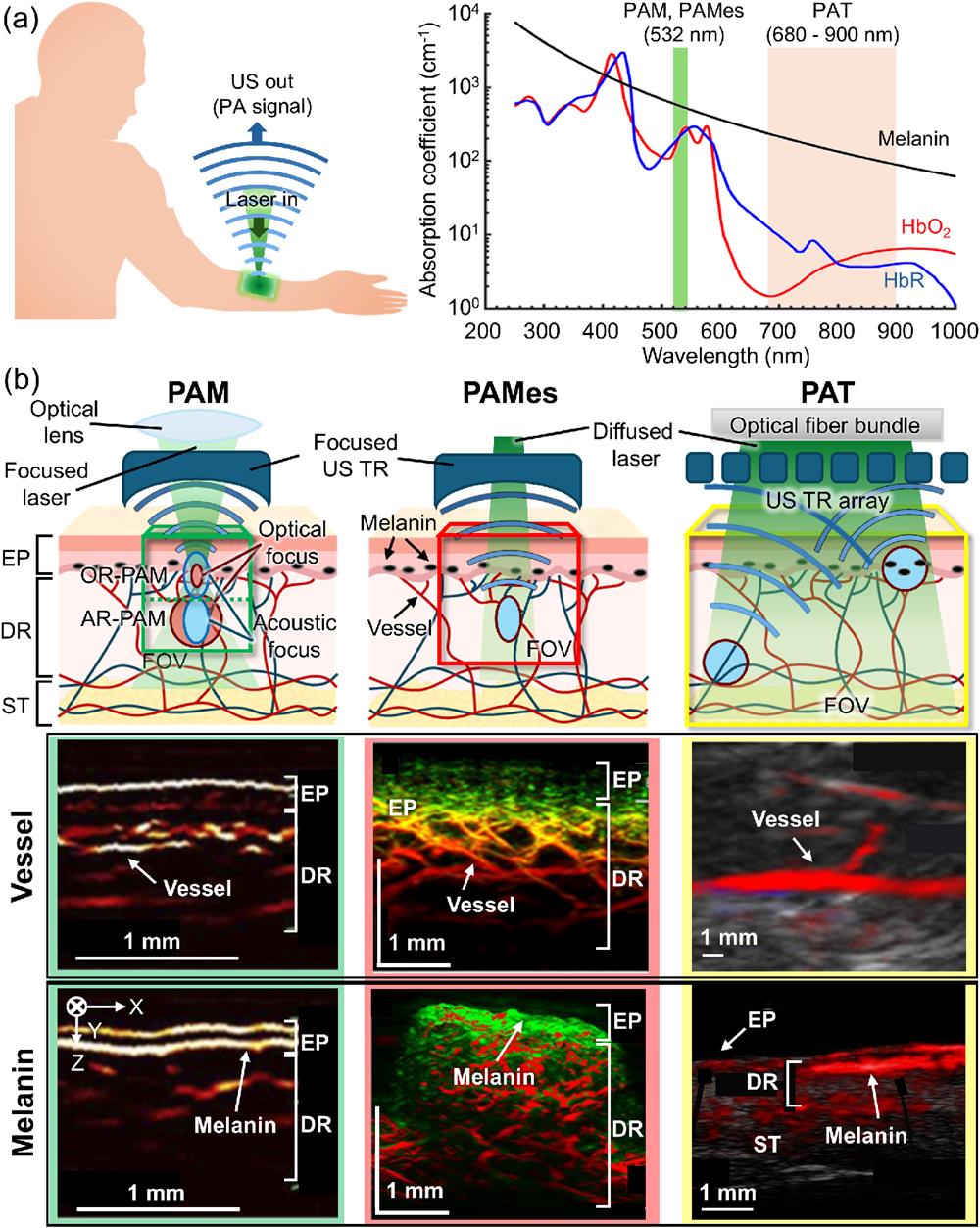
Set citation alerts for the article
Please enter your email address