
- Chinese Optics Letters
- Vol. 22, Issue 4, 040603 (2024)
Abstract
1. Introduction
Random lasers (RLs) are lasers that can be generated without conventional resonators. The light amplification of RLs is realized by multiple scattering feedback, for example, in laser crystals or amorphous powders[1,2]. Due to their low cost, compact size, and simple integration, RLs have received extensive research attention[3,4]. Among many RL systems, polymer fiber RLs possess unique advantages. Two-dimensional confinement from fiber waveguide geometry significantly enhances multiple scattering through total internal reflection, resulting in RLs with a lower threshold, directionality, and integrated simplicity. Furthermore, polymers effortlessly combine various gain materials and scattering structures by doping materials to control RLs. Researchers are highly interested in RLs based on polymer optical fibers (POFs) for these two reasons. Hu’s team first reported POF-based RLs in 2013[5], followed by demonstrations of polarized RLs using oriented POFs in 2016[6]. In addition, the team also demonstrated real-time temperature-controlled RLs based on POFs[7]. The stability and tunability of the RLs based on POF were demonstrated through these investigations, indicating their vast potential for use in sensors, optical switches, and speckle-free imaging.
Most RL research has focused on their frequency-domain characteristics. However, studying RLs in the time domain is also crucial, particularly for their dynamics[8–11]. In 2002, Cao’s team investigated the dynamic response of RLs and discovered that the emission pulse width narrows significantly when pump energy surpasses the lasing threshold[12]. Several subsequent works have explored short pulse generation and the time-domain characteristics of RLs. For instance, in a glass cuvette filled with a dye solution, the Alfano group achieved a 50 ps pulse width using a 230 µJ pumping[13]. Pecoraro et al. analyzed the time-emission characteristics of rhodamine-doped hybrid powder using photomultiplier tubes and found that the generation of RLs results in a narrower time distribution[14]. Shi et al. observed two inflection points in the temporal profile associated with the operating threshold and laser mode conversion as pump energy increased. They also observed a coherent RL’s pulse width of 5.03 ns in vertically pumped random silver nanowire–dye solution lasers at a pump energy of
Conventional lasers usually use gain-switching or
Sign up for Chinese Optics Letters TOC. Get the latest issue of Chinese Optics Letters delivered right to you!Sign up now
In this work, we generated picosecond and low-threshold POF RLs based on the gain-switching mechanism. First, three POFs doped with different scattering nano-particles (NPs) were prepared using the Teflon mold. Then, we employed the gain-switching mechanism to obtain stable RL pulse outputs in the picosecond range. Notably, the pulse widths of RLs are an order of magnitude narrower than that of pumped nanosecond pulses. Furthermore, we observed that the emission characteristics in the time domain were influenced by the polarization angle of the RLs, facilitating the study of amplified spontaneous emission (ASE) and RL emission dynamics. To our knowledge, this is the first analysis of the time- and frequency-domain properties of polymer fiber-based RLs and the first report of gain-switched picosecond RLs. Additionally, the pulse width of 0.6 ns is one of the shortest reported RL pulse widths. Our study introduces an innovative approach by introducing the gain-switching mechanism to picosecond RLs based on POFs, which has not been previously reported for RLs.
2. Results and Discussion
The gain medium and the scattering system are the key elements to constitute RLs. We doped the gain medium (the laser dye PM597), and the scattering nanoparticles into the core of POF to fabricate POF-based RLs. To evaluate the stability and reliability of the short-pulse RL, three different scattering systems were designed, wherein three distinct types of scattering particles were doped in the POF, namely, polyhedral oligomeric silsesquioxanes (POSS) NPs,
Size of Doped NPs (nm) | Core Size (µm) | Cladding Size (µm) | |
---|---|---|---|
POPOF | 200 | 26.7 | 696.4 |
FePOF | 10 nm Fe3O4@5 nm SiO2 | 59.5 | 637.2 |
AuPOF | 29 | 68.4 | 639.5 |
Table 1. Parameters of POFs
Figure 1.Optical micrographs of three types of disordered POF cross sections, corresponding to (a) POPOF, (b) FePOF, and (c) AuPOF; (d) experimental setup for testing RL emission.
The measurement setup is illustrated in Fig. 1(d). A
Figure 2 presents the RL emission spectra of POPOF, FePOF, and AuPOF at different pump energies in Figs. 2(a), 2(c), and 2(e), respectively. Additionally, Figs. 2(b), 2(d), and 2(f) show the integral intensities of Figs. 2(a), 2(c), and 2(e) at different pump energies, respectively. Because the phenomenon for the three kinds of POFs is similar, here we provide a detailed analysis of POPOF. Figure 2(a) shows broad fluorescence emission at low pump energy, which evolves into a narrow gain peak at the central wavelength of 568.2 nm when the pump energy exceeds the threshold. Furthermore, multiple sharp lasing peaks appear at the top of the emission peak, as customary for coherent RLs, to represent various laser modes originating from several closed-scattering loop cavities[23]. Figure 2(b) depicts the integrated emission intensities as a function of the pump energy for the polymer fiber RL system, where the nonlinear dependence of the emission intensity on the pump energy indicates lasing action. A noticeable inflection point is observed in Fig. 2(b), corresponding to the threshold of about 40.79 µJ. The threshold for FePOF and AuPOF is approximately 46.72 µJ and 72.95 µJ, respectively. The three types of random fiber lasers vary regarding the number of laser modes, the emission wavelength of the peaks, and the threshold value. The RL spectrum based on POPOF exhibits more peaks and the lowest threshold, since the doping concentration of POSS NPs is the highest, resulting in the smallest scattering mean free path and facilitating the generation of the RL. FePOF-based RL spectra contain fewer spikes and slightly higher thresholds than POPOF. Figures 2(a) and 2(b) demonstrate several modes in the random lasing spectrum, indicating the production of typical coherent RLs. AuPOF-based RLs, with the lowest doping concentration, demonstrate a noncoherent RL with fewer peaks on the top of its spectrum. It has the highest threshold compared with the other two RLs. This implies that the RL based on AuPOF does not form closed scattering loop cavities to form coherent RLs. Moreover, the picosecond RLs exhibit a lower threshold compared to conventional lasers due to their lack of a traditional resonator structure. The scattering cavity of the RL necessitates less energy for pumping than multiple reflections in traditional resonators. Furthermore, the disordered scattering is significantly amplified through total internal reflection owing to the two-dimensional confinement of the polymer waveguide geometry, reducing the threshold.
Figure 2.RL emission property of three POFs. The emission spectrum variations with pump energy of POPOF, FePOF, and AuPOF are shown in (a), (c), and (e), respectively. The variations of integral intensity with pump energy of POPOF, FePOF, and AuPOF are shown in (b), (d), and (f), respectively.
Figures 3(a), 3(c), and 3(e) are the normalized time distributions of POPOF, FePOF, and AuPOF, respectively, reflecting the process of RLs from the establishment to decay in the time domain. The pulse widths under different pump energy are shown in the second column. The time distribution curve fluctuates due to the rapid photodetector’s typical response[23]. The normalized time distribution was fitted with a Gaussian function, and Fig. 3(b) illustrates the link between the pump energy of the POPOF-based RL and the time distribution pulse width. The red dotted line represents the corresponding threshold in Fig. 2(b). The time signal starts to grow quickly when the pump light’s energy reaches 23 µJ. When the energy increases, the pulse width decreases from 6.4 ns at 23 µJ to 2.4 ns at 38 µJ, which is narrower than the pump pulse width of 6 ns. This phenomenon indicates that short-pulse RLs are beginning to build. The primary mode of PM597 emission dynamics is stimulated emission when the pump energy is significantly higher than the threshold. At pump energy of 50 µJ, the pulse width decreases to 0.6 ns, demonstrating the entire establishment of the short-pulse RLs. At pump energy of 50–65 µJ, the pulse width of the time pulse tends to be stabilized. Table 2 illustrates the variation of pulse width and decay time with pump energy for the three types of RLs. As the pump energy increases from below to above the threshold, the decay time and pulse width reduce by an order of magnitude. Specifically, the fall time of POPOF-based RL decreases from 19.9 ns at 23 µJ to 2.3 ns at 50 µJ. At sufficiently high pump energy, the pulse widths of all three types of POFs can be less than 1 ns. Although these three RLs have varying peaks and thresholds and produce distinct types of RLs owing to different scattering structures, they all eventually generate picosecond RL pulses, as evidenced by Figs. 2 and 3.
POF | Pump Energy (µJ) | Fall Time (ns) | Pulse Width (ns) |
---|---|---|---|
POPOF | 23 | 19.9 | 6.4 |
38 | 11.6 | 2.4 | |
50 | 2.3 | 0.6 | |
FePOF | 37 | 20.4 | 5.8 |
40 | 8.6 | 1.5 | |
45 | 2.1 | 0.7 | |
AuPOF | 65 | 18.4 | 6.6 |
80 | 12.8 | 2.4 | |
90 | 3.4 | 0.7 |
Table 2. Single Exponential Decay Fitting of Temporal Properties
Figure 3.RL pulse characteristic of polymer fibers. The normalized time distributions for POPOF, FePOF, and AuPOF are shown in (a), (c), and (e), respectively. The emission pulse widths of POPOF, FePOF, and AuPOF are shown in (b), (d), and (f), respectively. The red dotted line represents the threshold for the random lasing emission spectrum.
Upon excitation by a pump laser pulse, the laser dye molecules undergo a transition from the ground state to the excited state. This results in an exponential growth of populations at the upper energy level and a rapid increase in fluorescence form in the time domain followed by a gradual decline. The competition between RL gain and loss is dependent on gain saturation, which is influenced by the pump pulse energy; higher pump energy yields more gain than loss, and vice versa. The gain medium is stimulated until population inversion reaches the threshold; at this point, the gain switch is activated, allowing a large amount of optical energy to flow through the gain medium. Consequently, there is a sudden increase in population inversion and gain. When the continuous pump light intensity approaches the threshold level, the pump pulse light superimposed on laser dye molecules causes the number of reversing particles in the “scattering cavity” to rapidly exceed the threshold. This leads to an increase in excited radiation output, generating a picosecond random laser pulse. The rate equation is used to describe the time-dependent gain
Figure 4.(a) Simulation of gain and RL pulse compression. The red line is the gain curve of the RLs. The blue line is the pump laser pulse. The green line is the fluorescence pulse below the threshold. The black line is the RL pulse. (b) Simulation of pulse width variation when pump energy is below and above the threshold.
The ordinary differential Eq. (1) is solved using the Runge–Kutta method[25]. When the pump energy increases, the value
We analyzed the normalized intensity distribution of picosecond pulses of RLs based on POFs. Figure 5(a) demonstrates that the repetition rate of the RL based on POFs is identical to that of the pump laser, which is 10 Hz, indicating gain-switched pulsed laser characteristics. We utilized the dual channels of the oscilloscope to capture the pulses of both random and pump lasers, as illustrated in Fig. 5(b). The delay time of the random laser pulses compared to the pump laser pulses is 2 ns when the pump energy is 50 µJ, implying rapid activation of the gain switch in the POF-based RL. In conventional lasers, short pulses are generated via gain switching by modulating the pump power.
Figure 5.(a) Normalized periodic distribution of short pulse RLs within 1 s. Inset, an enlarged view of an RL pulse. (b) Simultaneous acquisition of pump laser and RL pulses.
In Fig. 6(a), we pumped POPOF, FePOF, and AuPOF with energies of 50, 45, and 90 µJ, respectively. It was observed that the pulse widths of all the RLs based on POF remained stable between 0.6 and 0.8 ns after 35,000 consecutive pumping pulses. This indicates the stability of the picosecond RLs over a long period of pumping time. The small fluctuations in pulse width were caused by 4% fluctuations in the pump laser energy. In Fig. 6(b), we investigated the relationship between the polarization direction of RLs and their pulse width. We positioned a polarizer in front of the photodetector and aligned it with the polarization direction of the pump laser. We define the rotation angle of the polarizer at this time to be 0°. By changing the angle
Figure 6.(a) Linewidth of the three POFs at 35,000 pumps; (b) the pulse width of RLs varies with the angle θ.
3. Conclusion
In conclusion, gain-switched RLs with a pulse width of 0.6 ns are generated using specially designed POFs. The lowest threshold is reached at a minimum of 40.79 µJ. The effectiveness and reliability of the gain-switching mechanism in POF-based RLs are demonstrated via three different scattering regimes. Stable short-pulse RL emission is observed, demonstrating consistency with the pump pulse frequency. The effect of polarization angle on RL duration is also investigated, proving that the emitted light is a mixture of RL and ASE light. Gain-saturation model simulations confirm the narrowing phenomenon of the RL pulse relative to the pump pulse, shedding insight into the gain-switching dynamics of polymer fiber-based RL. This work obtains one of the shortest pulses of RL and offers a new perspective on short-pulse RL generation and its emission dynamics.
References
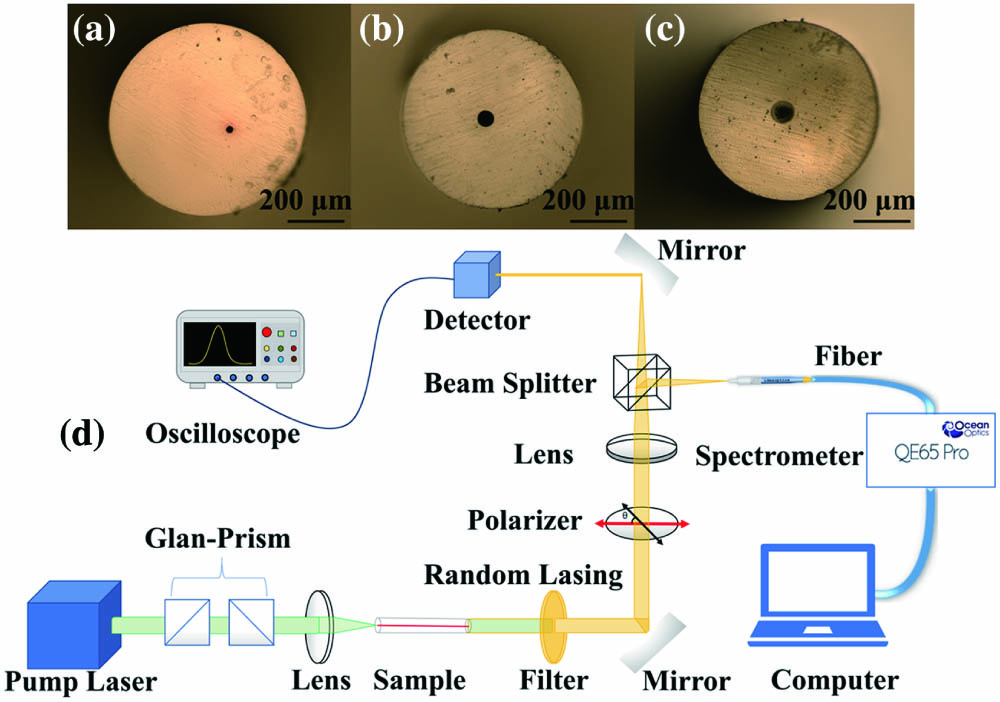
Set citation alerts for the article
Please enter your email address