
- Matter and Radiation at Extremes
- Vol. 9, Issue 3, 037403 (2024)
Abstract
I. INTRODUCTION
The composition of the Earth has long been of wide interest and has been a topic of significant scientific research. The relative absence of Xe from the Earth’s atmosphere compared with its chondritic abundances and the anomalous ratios of 3He/4He in the Earth’s mantle1,2 suggest the presence of He and Xe reservoirs in the Earth’s interior. Early studies hypothesized that Xe might have escaped from the atmosphere3,4 or be stored in the interior of the Earth;5,6 meanwhile, He was predicted to be trapped in compounds stabilized in the lowermost mantle regions. Solving these prominent geoscience puzzles requires finding stable structures and understanding the physics and chemistry of Xe/He-bearing compounds under high pressure–temperature (P–T) conditions, which is challenging because both He and Xe have full-shell electronic structures and tend to be unreactive. Only a small number of Xe compounds have been synthesized under ambient conditions.7–9 Pressure is a clean and effective tool to tune electronic properties10 and can often increase chemical reactivity.11 A slew of He and Xe compounds have been synthesized or predicted at high pressures.6,12–23
On the geoscience research front, attempts to incorporate Xe into ices, clathrates, and sediments of the Earth’s crust were not successful.24–26 Later experiments found trapped Xe in quartz27 and predicted stable xenon oxides,13,28 but these oxides are unstable in equilibrium with metallic iron in the lower mantle, and xenon silicates may decompose spontaneously in the lower mantle.28 Theoretical studies show the possible presence of Xe trapped in the Earth’s core in the form of stable Fe–Xe compounds,6 and Fe and Xe may form solid solutions.17,29 Recently, He and Xe have been predicted to react with FeO2 with the formation of compounds that are thermodynamically stable under deep-Earth conditions.30,31
There is a lack of Xe/He crystalline compounds (not He inclusions in crystals) reported to date as being thermodynamically stable under the P–T conditions corresponding to the great depths near to or inside the core of the present Earth, where pressures rise above 130 GPa and temperatures are higher than 3000 K. Study of the relevant compounds may provide insight into the geological trapping of He and Xe. It should be noted that several studies have suggested that halogen concentrations may actually not be as low as previously thought in chondrites,32–36 which motivates us to search for stable Xe/He iron halides crystal compounds at high pressures.
In this work, we show by extensive computational study that Xe/He-bearing iron halides can be stabilized at moderate pressures below 60 GPa over wide ranges of temperature relevant to diverse geological environments. Our crystal structure searches in conjunction with first-principles energetic calculations and molecular dynamics simulations establish pertinent P–T phase diagrams and demonstrate the thermodynamic stability of several Fe–F–He, Fe–F–Xe, and Fe–Cl–Xe compounds that could have existed in the interior of the early Earth. These findings introduce a new paradigm for sequestration of He and Xe inside the Earth by establishing a critically needed connection to the primordial origin of the geological trapping of these elements, making possible the scenarios of previously proposed Xe/He-bearing compounds that are only viable at much greater depths in the lowermost mantle or core regions of the present Earth.
II. RESULTS
Our crystal structure search employs a global free-energy optimization algorithm as implemented in the CALYPSO methodology37,38 in conjunction with first-principles calculations to determine energetic and dynamic structural evolution under wide-ranging P–T conditions. The different components of the compounds in this study are shown in Table S1 (
Figure 1.(a)–(c) Ternary phase diagrams of the Fe–F–He, Fe–F–Xe, and Fe–Cl–Xe systems at selected pressures and 0 K. Five thermodynamically stable ternary compounds have been identified. (d) Composition–pressure phase diagram of the five ternary compounds over a wide range of pressure and at 0 K.
Figure 2.Crystal structures of stable ternary compounds: (a)
Of the two He-bearing compounds, FeF2He crystallizes in the AlCu2Mn (Heusler) structure type in Fm-3m symmetry, adopts a face-centered-cubic lattice, and comprises FeF8 units that host He atoms. In this structure, the FeF8 hexahedrons are connected to each other via common edges, and He atoms sit between the hexahedrons. At 60 GPa, the Fe–F, Fe–He, and F–He nearest distances take uniform values of 2.07, 2.39, and 2.07 Å, respectively. Meanwhile, FeF3He in P63/m symmetry comprises FeF9 units and He atoms in otherwise similar structural arrangements, where the Fe–F, Fe–He, and F–He nearest distances are 2.02, 3.03, and 1.98 Å, respectively. There are three Xe-bearing compounds, namely, FeF2Xe in P21/m symmetry, FeF3Xe in Pmmn symmetry, and FeCl3Xe in P63/mmc symmetry. The P21/m-FeF2Xe comprises FeF6 units arranged in planar structures with the Xe atoms positioned between the planes, and the nearest Fe–F distances in this phase are 1.94–2.11 Å, while the Fe–He and F–Xe nearest distances are 2.69 and 2.67 Å, respectively. In Pmmn-FeF3Xe, each Fe atom is surrounded by eight F atoms, and the FeF8 decahedron units form a planar structure by sharing the same edges, where Fe–F nearest distances are in the range of 1.96–2.05 Å, while the Fe–Xe and F–Xe nearest distances are 2.69 and 2.59 Å, respectively. The P63/mmc-FeCl3Xe contains FeCl6 units, which are arranged in chains that share the same plane, and the Fe–Cl, Fe–Xe and F–Xe nearest distances are 2.21, 3.62, and 2.93 Å, respectively.
We also performed Bader charge analysis on the predicted ternary compounds, as shown in Table S4 (
To assess the geological viability of the predicted Xe/He-bearing compounds, it is essential to establish their P–T phase diagrams under the conditions corresponding to the interiors of both the early and present Earth. To this end, we have performed two sets of calculations. We first computed the phonon dispersions and the corresponding phonon densities of states of all the predicted structures and associated binary and elemental systems, and then used the results as input to evaluate the vibrational contribution to the entropy. Combining this with the total internal energy, pressure, and volume determined by first-principles calculations, we obtained the Gibbs free energies for FeF2He, FeF3He, FeF2Xe, FeF3Xe, and FeCl3Xe, along with the results for the related binary and elemental crystals, which allow a systematic determination of phase boundaries separating the ternary compounds from the decomposed constituent binary iron halides and Xe/He. Additionally, we performed ab initio molecular dynamics (AIMD) simulations over wide P–T ranges to evaluate the temperature-driven diffusive and melting behaviors, which further characterize the distinct states of matter of the Xe/He-bearing ternary compounds under the P–T conditions encountered in widely changing geological environments.
On the basis of Gibbs free energy calculations and AIMD simulations, we have constructed the P–T phase diagrams for the five Fe–F–He and Fe–X–Xe (X = F, Cl) compounds (Fig. 3), which show that these compounds become thermodynamically stable against binary and elemental decompositions over large swaths of P–T space, starting at moderate pressures. Rising temperature helps to stabilize most of the examined ternary compounds at reduced pressures, indicating favorable entropic contributions to the structural stabilization, with the exception of FeF3He, which requires higher pressures for stabilization at rising temperatures, indicating a pressure-dominated stabilizing mechanism as previously seen in FeO2He. Overall, all the ternary compounds possess stability fields that are compatible with the P–T conditions inside the early Earth, and this finding offers a plausible scenario for the sequestration of He and Xe in the initial stages of Earth’s differentiation when the volatile elements were separated and redistributed from the primordial chondritic contents. The stability fields of these ternary compounds extend to higher P–T regions coinciding with the geotherm of the present Earth, enlarging considerably the regions in the Earth’s interior where Xe/He-bearing compounds can exist in stable nongaseous forms, which has major implications for interpreting the origin and cycling derived from the geochemical analysis of mineral assemblages entrained from distinct mantle regions. For example, primordial He observed in plumes at volcanic hot spots has been taken as a key evidence of its entrainment from the deep lower mantle,45 yet the present results indicate that the minerals containing primordial He could have come from much shallower depths near the top of the lower mantle, allowing fresh interpretation of the dynamic evolution of the Earth’s interior.
Figure 3.Pressure–temperature (
The results of our AIMD simulations further reveal an interesting phenomenon of partial melting of the ternary compounds as the temperature rises above certain threshold values, characterized by the onset of an intriguing partial melting state where the lighter-element sublattice melts, with the corresponding constituent atoms turning diffusive while the remaining crystal frame remains intact in the solid phase. As the temperature increases further, the entire crystal transitions into a liquid phase where all the constituent atoms of the entire crystal becomes diffusive. This scenario is illustrated for the representative cases of FeF3He, FeF3Xe, and FeCl3Xe in Fig. 4 and Fig. S6 (
Figure 4.(a)–(c) Mean-square displacements (MSDs) of atoms in
III. DISCUSSION
During the initial phase of its differentiation process, the early Earth is considered to have harbored P–T conditions up to 60 GPa–3000 K,46 and the phase diagrams in Fig. 3 thus indicate that the sublattice melting and liquid phases of the iron–halide–Xe/He compounds are viable constituents deep inside the planet during the crucial initial phase of its differentiation process, along with the corresponding solid phases in the outer layers with lower P–T conditions during core–mantle separation. Moreover, since the stability fields of these ternary compounds extend from the top to the bottom of the lower mantle of the present Earth, primordial He and Xe can exist in solid, partial melting solid, or liquid phases in the entire vast volume of Earth’s lower mantle either as stable deposits or as part of the dynamic mineral cycling process.
The present study also sheds light on the anomalously low terrestrial abundance of halogens relative to chondritic meteorites.47 Previous studies40 proposed that halogens may be sequestered in the Earth’s mantle and core via the formation of iron halides. Our results suggest a new mechanism for halogen sequestration via halogen-bearing Fe–X–Xe/He (X = F, Cl) compounds that can be stabilized under moderate P–T conditions corresponding to those inside the early Earth and also in the present Earth’s vast lower mantle. These results provide an additional material platform to account for the terrestrial halogen distribution that holds the key to understanding geological evolution during the Earth’s formation and subsequent differentiation and accretion.
In conclusion, through an extensive crystal structure search in conjunction with first-principles energetic calculations, we have identified a series of Xe/He-bearing iron halides FeF2He, FeF3He, FeF2Xe, FeF3Xe, and FeCl3Xe that are thermodynamically stable at moderate pressures below 60 GPa over wide ranges of temperatures and are thus viable inside the early Earth during its initial stages of differentiation. This discovery offers insights into the long-standing puzzle of terrestrial abundance anomalies of He and Xe that have their roots in the earliest geophysical and geochemical evolutions of the Earth, and expands considerably the range of existence of Xe/He-bearing compounds in the present Earth. Moreover, AIMD simulations reveal partial melting of these iron–halide–Xe/He compounds at rising temperatures, resulting in intriguing sublattice melting states with the lighter elements in diffusive modes inside the remaining solid crystal framework, before transitions into the liquid states occur at further increased temperatures. These findings make a compelling case for sequestration of He and Xe in the early Earth, thereby establishing a crucial but hitherto missing link to the presence of these elements inside the present Earth as suggested by extensive existing studies. The present results also have broad implications for interpretation and elucidation of geophysical and geochemical processes that are sensitive to the origins of such minerals entrained from distinct deep-Earth localities, which offer insights into the evolutionary dynamics and mineral cycling inside the Earth.
SUPPLEMENTARY MATERIAL
ACKNOWLEDGMENTS
Acknowledgment. This work is supported by the National Natural Science Foundation of China (Grant Nos. 12204280 and 12147135), the Postdoctoral Science Foundation of China (Grant No. 2021M691980), Natural Science Foundation of Shandong Province (Grant No. ZR202103010004), the Jilin Province Science and Technology Development Program (Grant No. YDZJ202102CXJD016), the Program for Jilin University Science and Technology Innovative Research Team (2021TD-05), and the Program for Jilin University Computational Interdisciplinary Innovative Platform. We used the computing facility at the High-Performance Computing Center of Jilin University.
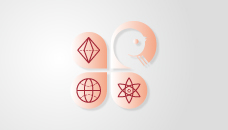
Set citation alerts for the article
Please enter your email address