
- Chinese Optics Letters
- Vol. 22, Issue 8, 081202 (2024)
Abstract
Keywords
1. Introduction
From the physics of elementary particles to the chemistry of life, chirality is an essential property in modern science and occurs widely in physical structures such as DNA, sugars, and amino acids[1–4]. Chiral molecules are molecules with mirror images that cannot be superimposed on each other, called enantiomers, and are characterized by extremely different biological activities and toxicities[5,6]. For example, limonene with different handedness has a different smell, which has important consequences, as in the case of the fruit fly pheromone Olean, where one-handedness attracts females and the other attracts males[7]. Moreover, in the pharmaceutical industry, chirality plays a vital role in which one enantiomer may act as a drug and another enantiomer can be inactive or even lead to serious side effects[8–11]. For example, thalidomide, often mentioned as a therapeutic example, provides an effective relief medication for morning sickness in pregnant women, but the mirror image causes severe birth defects. Therefore, the ability to discern chirality with high sensitivity and high speed in the pharmacokinetics and the pharmacodynamic profiles is highly desirable. The chiroptical effect is a special property of chiral materials in that the optical responses to the left-handed (LCP) and right-handed circularly polarized (RCP) light are different[12,13]. Thus, circular dichroism (CD) and optical rotation (OR) can be utilized to distinguish chiral systems, in which CD is induced by different extinction coefficients[14–16] and the OR is based on the different phase velocities for LCP/RCP light[17–19]. Moreover, high-performance liquid chromatography (HPLC) is characterized by high-resolution chirality detection owing to chiral solid phase separation[20]. However, biomolecular applications of these methods are hindered by the complex sample preparation and the high cost of chiral columns. In addition, the metabolic processing of chiral molecules and enantiomer drugs cannot be quickly monitored in cell and animal experiments due to the lack of sensitivity for the chiroptical effect and the complexity of HPLC. Therefore, it is important to develop a method that can overcome the drawbacks and better monitor chiral molecular metabolism in life.
The metal cladding optofluidic chip (MCOC) possesses a variety of fascinating optical properties[21–25]. The unique feature is the excitation of ultrahigh-order guided modes (UOMs) in the cavity under illumination. Moreover, the reflective coefficient of UOMs is extremely sensitive to the dielectric coefficient of the guided layer. For the MCOC, the reduced dispersion equation for the mth-order eigenmode can be written as
Based on these properties, we demonstrated the discrimination of two pairs of chiral molecules in cell and animal experiments. As shown in Fig. 1(a), the intensity of LCP/RCP light shows a difference in reflective light when the chiral molecule is injected into MCOC due to the different dielectric coefficients of the chiral molecules for LCP and RCP. The difference in reflectivity of LCP/RCP, D, can be expressed as (see more details in Supplementary Material Section I)
Figure 1.Schematic of the MCOC and the discerning process. (a) The MCOC consists of a coupling layer, a guided layer (containing cavity), and a base layer. When chiral molecules with different chirality are injected into the cavity, the reflected beam is altered. For example, the R-limonene molecules exhibit high reflectivity for left-handed circularly polarized lasers, while S-limonene exhibits high reflectivity for right-handed circularly polarized lasers. (b) Theoretical results of the difference in reflectivity.
2. Results
To demonstrate the ability to distinguish chiral molecules, we measured the difference in reflectivity of prepared methotrexate (MTX) and amlodipine (ADP) molecule solutions. The results showed that the difference in reflectivity of two pairs of chiral molecules is proportional to the concentration with the opposite sign coefficient, which is consistent with theoretical predictions. The experimental setup is shown in Fig. 2(a). The power and spin angular momentum (SAM) of the laser beam (generated by a 100 mW 532 nm laser) are controlled by a combination of a half-wave plate (HWP), quarter-wave plates (QWPs), and a linear polarizer (LP). Two photoelectric detectors (PDs) are used to detect the incident and reflective beam intensities. The samples of the prepared chiral molecule solution are injected into the MCOC with a microsyringe system. Then, the reflectivity of LCP/RCP is detected to generate the difference in reflectivity. After each experiment, a blank buffer was used to wash the cavity. Figures 2(b) and 2(c) show the experimental results of the prepared chiral molecule solution, in which the difference in reflectivity is positive and negative for R-structure molecules (R-MTX and R-ADP) and S-structure molecules (S-MTX and S-ADP), respectively. Its absolute value increased linearly with the concentration of chiral molecules, with correlation coefficients of 0.0044 ± 0.0002/−0.0046 ± 0.0003 mL · mg−1 for R-/S-ADP and 0.0030 ± 0.0001/−0.0032 ± 0.0001 mL · mg−1 for R-/S-MTX. Therefore, the experimental results of the prepared chiral molecule solution are consistent with the theoretical results. Moreover, the changing intensity of the light induced by the optical rotation for the ADP is
Figure 2.Experimental setup and the results of the prepared chiral molecule solution. (a) Schematic of the experimental setup, which consists of a combination of a half-wave plate (HWP) and a linear polarizer (LP), quarter-wave plates (QWPs), photoelectric detectors (PDs), MCOC, and a microsyringe system. (b), (c) Experimental results of R-/S-MTX and the R-/S-ADP molecule solution with different concentrations.
In the following section, we will use the experimental results shown in Figs. 2(b) and 2(c) as the calibration data to detect the changing concentrations of different chiral R/S-MTX and R/S- ADP in the cell culture experiment and animal experiments, respectively.
The application of different concentrations of chiral drugs and treatment times has varied influences on cell proliferation. First, B16 cells were co-incubated with different concentrations of chiral drugs (S-MTX or R-MTX) for 24, 48, and 72 h. As shown in Fig. 3(b), the CCK-8 assay revealed that the chiral drug S-MTX had an obvious inhibitory effect on cell proliferation in the concentration range from
Figure 3.Effect of chiral MTX on cell growth and cell viability. (a) B16 (XX) cells were cultured with S-/R-MTX at a concentration of 100 µmol·L-1. After 1, 2, 3, 6, 12, and 24 h of culture, the culture medium and intracellular fluid were sampled to detect changes in the concentration (see Section 4). (b) Varying cell viability of B16 cells co-incubated with different concentrations of chiral drugs (S-MTX or R-MTX) at 24, 48, and 72 h; (c) fluorescence microscopy observation of B16 cells stained with AM/PI after incubation with chiral drugs for 3, 6, and 12 h; (d) various concentrations of S-/R-MTX in culture medium with different culture durations.
After adding chiral drugs and incubating for various durations, B16 cells were stained with AM/PI for fluorescence microscope observation, as shown in Fig. 3(c). Strong green fluorescence of living B16 cells was observed in the control group. Similarly, the B16 cells incubated with chiral drugs for 3 h remained alive and looked similar to those in the control group. When the incubation time was extended to 6 h, the green fluorescence of B16 cells decreased, and red fluorescence began to appear. After incubation for 12 h, the red fluorescence appeared in abundance, while the green fluorescence decreased significantly. In addition, the number of intact cells in the visual field was reduced, which means that the growth of cells was significantly suppressed. Moreover, observing cell morphology under an inverted microscope at different time points showed that after the application of chiral drugs, cells fused, and some cells became round and floated, which showed a significant difference between S-MTX and R-MTX at 6 h. With increasing time, the cell membrane broke and thickened, and a large number of floating cell fragments appeared, especially in the S-MTX group (see more details in Supplementary Material Section III). These phenomena are consistent with previous results, confirming that MTX has an inhibitory effect on B16 cells and that large variability exists between different chiral drugs.
Figure 3(d) presents the changes in S-/R-MTX concentrations in a culture medium, showing that the concentration of S-MTX decreased faster than that of R-MTX. The concentration decreased quickly after the addition of chiral drugs, and the absorption rate gradually decreased as the duration increased. Ultimately, the concentration in culture medium tended toward stability. Moreover, different concentrations of S-/R-MTX in a culture medium can be detected by our device, indicating the ability to perform monitoring.
Figure 4(a) shows the preparation of the samples in the hypertension model (see more details in Supplementary Material Section IV). A hypertension model was successfully established via induction with L-NAME in Wistar rats after 3 weeks, which can be proven by systolic/diastolic blood pressure over 140/90 mmHg compared to normotensive rats. The effect of the administration of chiral ADP on hypertensive rats is shown in Fig. 4(c). The results showed that management with S-ADP (
Figure 4.Preventive effect of chiral ADP on hypertension. (a) Wistar rats were dosed with L-NAME for 3 weeks to establish a hypertension model. After that, they were divided into three groups, the control group, the S-ADP group, and the R-ADP group, which were administered blank buffer, S-ADP solution, and R-ADP solution, respectively. Then, we collected blood samples in different periods and detected their chiral ADP concentrations. (b) Changing R-/S-ADP concentration in the blood after tail vein injection; (c) changes in blood pressure and heart rate in the S-ADP group; (d) changes in blood pressure and heart rate in the R-ADP group.
Figure 5.Contrast between the results of S-ADP and R-ADP.
Figure 5 shows the contrast between the results of S-ADP and R-ADP, in which S-ADP and R-ADP are different in the inhibition of hypertension, and S-ADP has a significant suppressive effect on hypertension. These results are consistent with the measured change in ADP concentration.
3. Conclusion
In conclusion, we proposed a new method to discern chiral enantiomers based on MCOC. The properties of our device were demonstrated both in cell and animal experiments, and the concentration of two pairs of chiral drugs was detected experimentally. As shown in Table 1, the proposed method realizes high sensitivity and a simple system structure, making it ideal for applications that require high-sensitivity chirality detection with simple and miniaturized devices. With our device, the changing concentrations of chiral drugs can be quickly monitored by all-optical means without complex sample preparation and high-cost chiral columns, which offers a novel method for achieving crucial chirality discrimination for pharmacokinetics and pharmacodynamics and presents opportunities in indicating the health status of humans.
Ref. | Structure | Mechanism | Accuracy |
---|---|---|---|
Ours | MCOC | UOMs resonance | 0.245 mmol/L |
[ | Ultraviolet–circular dichroism | Circular dichroism | 4.31 mmol/L |
[ | Hollow-center optical fiber | Optical rotation | 0.3 mmol/L |
[ | Chiral molecules split light | Reflection and refraction | 4.89 mmol/L |
[ | Continuous-wave cavity-enhanced polarimetry | Optical rotation | 1.52 mmol/L |
Table 1. Comparison of Other Optical Chirality Detection Methods
4. Methods and Materials
For analysis of cell viability, B16 cells were seeded on 24-well plates (
References
[2] V. Goldanskii, V. V. Kuzmin. Chirality, and cold origin of life. Nature, 352, 114(1991).
[5] A. J. Hutt, S. C. Tan. Drug chirality and its clinical significance. Drugs, 52, 1(1996).
[6] L. A. Nguyen, H. He, C. Pham-Huy. Chiral drugs: an overview. Int. J. Biomed Sci., 2, 85(2006).
[8] M. E. Franks, G. R. Macpherson, W. D. Figg. Thalidomide. Lancet, 363, 1802(2004).
[19] A. L. Nafie. Applications of Vibrational Optical Activity(2011).
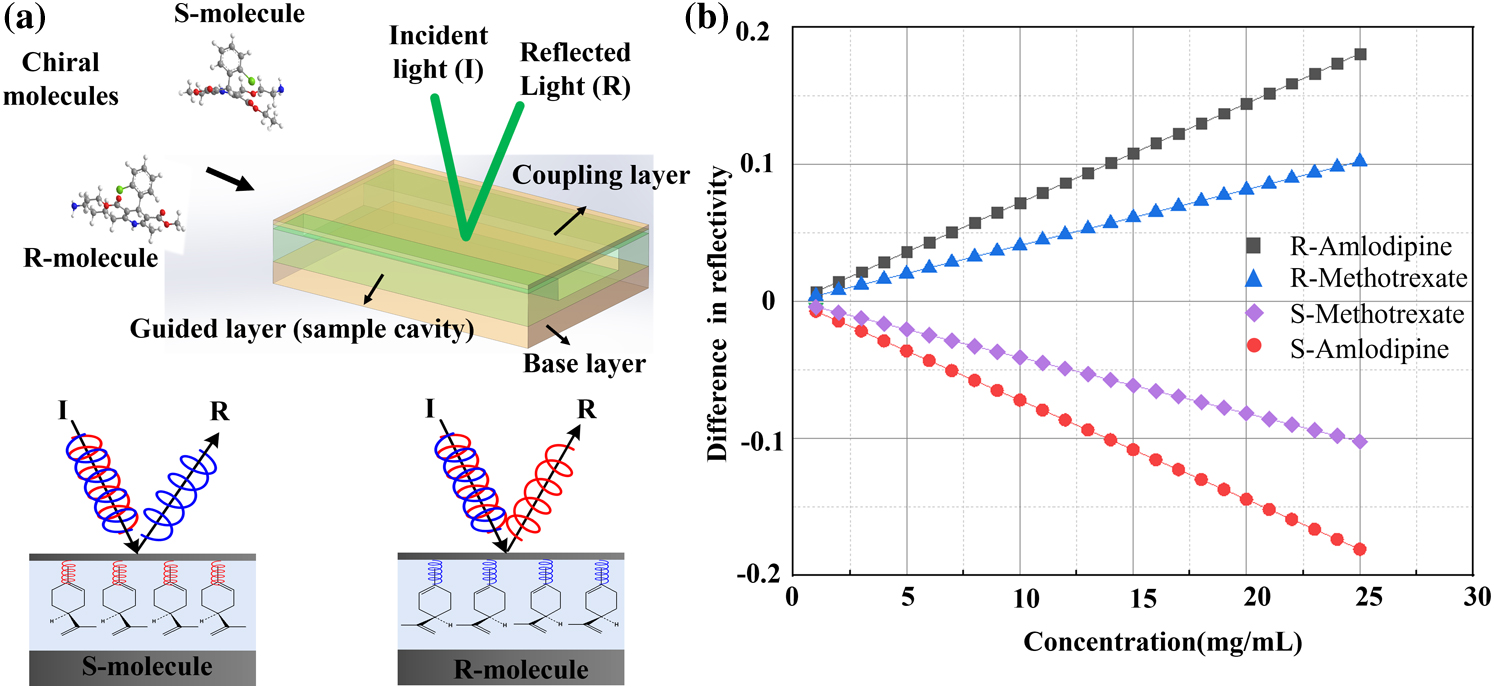
Set citation alerts for the article
Please enter your email address